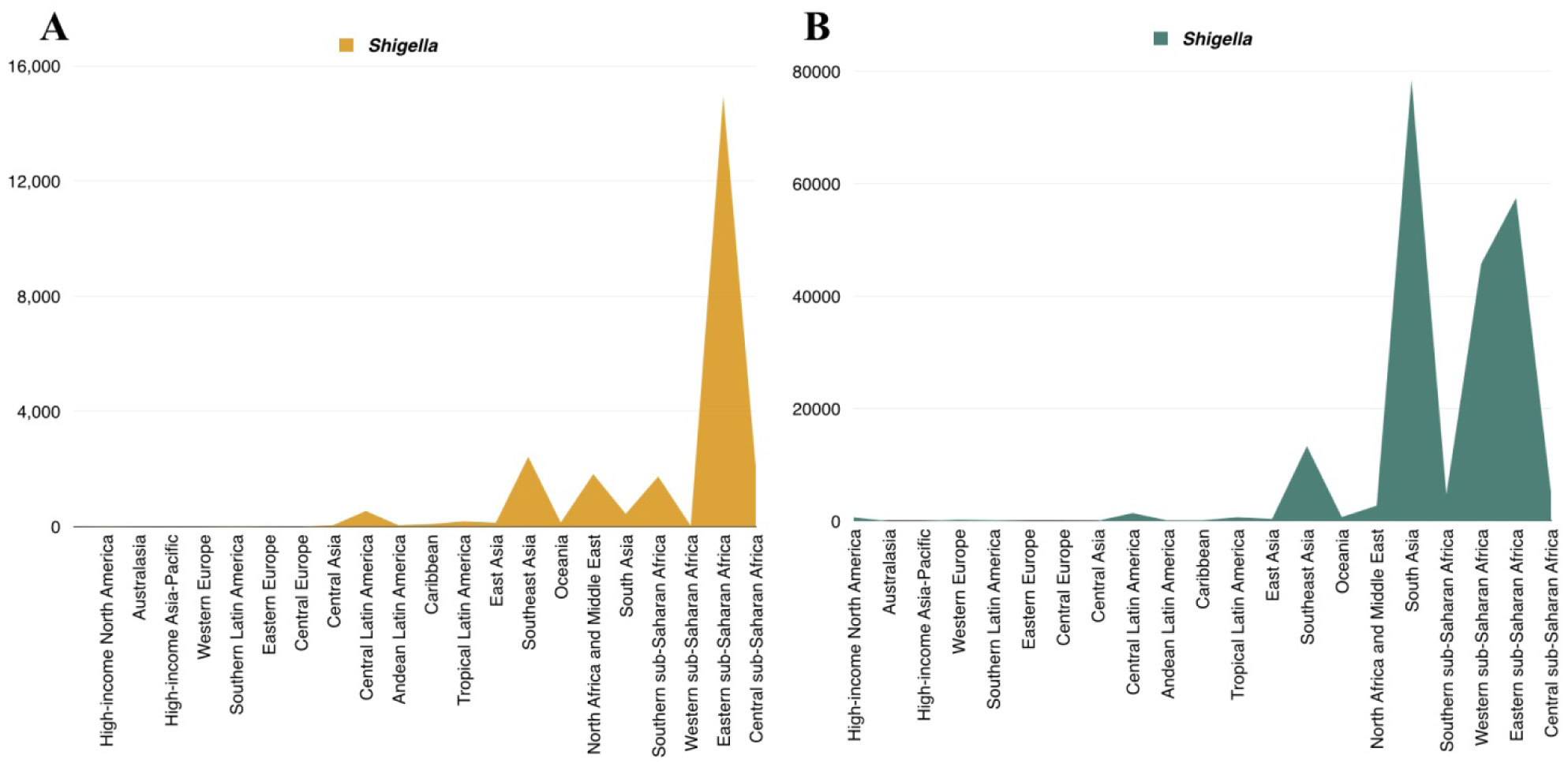
Citation: Sarmishta Mukhopadhyay, Sayak Ganguli, Santanu Chakrabarti. Shigella pathogenesis: molecular and computational insights[J]. AIMS Molecular Science, 2020, 7(2): 99-121. doi: 10.3934/molsci.2020007
[1] | Seyedeh Fahimeh Razavi, Leila Zarandi Miandoab, Elaheh Zadeh Hosseingholi, Nader Chaparzadeh . Radical scavenging capacity of RuBisCO bioactive peptides derived from Dunaliella salina and Spirulina platensis: An in silico and in vitro study. AIMS Molecular Science, 2025, 12(1): 49-66. doi: 10.3934/molsci.2025004 |
[2] | Jean Emmanuel Mbosso Teinkela, Philippe Belle Ebanda Kedi, Jean Baptiste Hzounda Fokou, Michelle Isaacs, Lisette Pulchérie Yoyo Ngando, Gaelle Wea Tchepnou, Hassan Oumarou, Xavier Siwe Noundou . In vitro anti-trypanosomal activity of crude extract and fractions of Trichoscypha acuminata stem bark, Spathodea campanulata flowers, and Ficus elastica lianas on Trypanosoma brucei brucei. AIMS Molecular Science, 2024, 11(1): 63-71. doi: 10.3934/molsci.2024005 |
[3] | Praveen Kumar Neela, Rajeshwari B.V, Mahamad Irfanulla Khan, Shahistha Parveen Dasnadi, Gosla Srinivas Reddy, Akhter Husain, Vasavi Mohan . Genetic influences on non-syndromic cleft lip palate: The impact of BMP4, RUNX2, PAX7, and TGFB3 allelic variations. AIMS Molecular Science, 2024, 11(4): 322-329. doi: 10.3934/molsci.2024019 |
[4] | Yutaka Takihara, Ryuji Otani, Takuro Ishii, Shunsuke Takaoka, Yuki Nakano, Kaori Inoue, Steven Larsen, Yoko Ogino, Masashi Asai, Sei-ichi Tanuma, Fumiaki Uchiumi . Characterization of the human IDH1 gene promoter. AIMS Molecular Science, 2023, 10(3): 186-204. doi: 10.3934/molsci.2023013 |
[5] | Anna Kapambwe Bwalya, Robinson Mugasiali Irekwa, Amos Mbugua, Matthew Mutinda Munyao, Peter Kipkemboi Rotich, Tonny Teya Nyandwaro, Caroline Wangui Njoroge, Anne Wanjiru Mwangi, Joanne Jepkemei Yego, Shahiid Kiyaga, Samson Muuo Nzou . Investigation of single nucleotide polymorphisms in MRPA and AQP-1 genes of Leishmania donovani as resistance markers in visceral leishmaniasis in Kenya. AIMS Molecular Science, 2021, 8(2): 149-160. doi: 10.3934/molsci.2021011 |
[6] | Abdulrahman Mahmoud Dogara, Ateeq Ahmed Al-Zahrani, Sarwan W. Bradosty, Saber W. Hamad, Aisha Abdullahi Mahmud, Hussain D. Almalki, Mustapha Abdullahi, Abubakar Abdullahi Lema, Hasan Nudin Nur Fatihah . In-vitro biological activity and in-silico studies of some volatile phytochemicals from the ethanol extract of Eugenia uniflora. AIMS Molecular Science, 2024, 11(3): 303-321. doi: 10.3934/molsci.2024018 |
[7] | Jin Hwan Do . Genome-wide transcriptional comparison of MPP+ treated human neuroblastoma cells with the state space model. AIMS Molecular Science, 2015, 2(4): 440-460. doi: 10.3934/molsci.2015.4.440 |
[8] | Kabyashree Phukan, Devid Kardong . Isolation of naringinase producing soil bacteria from Psidium guajava L. and Terminalia chebula Retz and its enzymatic activity. AIMS Molecular Science, 2020, 7(3): 292-304. doi: 10.3934/molsci.2020014 |
[9] | Irene Mwongeli Waita, Atunga Nyachieo, Daniel Chai, Samson Muuo, Naomi Maina, Daniel Kariuki, Cleophas M. Kyama . Differential expression and functional analysis of micro RNAs in Papio anubis induced with endometriosis for early detection of the disease. AIMS Molecular Science, 2020, 7(4): 305-327. doi: 10.3934/molsci.2020015 |
[10] | Patrizia A d'Alessio, Marie C Béné, Chantal Menut . d-Limonene challenging anti-inflammatory strategies. AIMS Molecular Science, 2022, 9(2): 46-65. doi: 10.3934/molsci.2022003 |
Diarrheal diseases have always been a threat to growing human population all over the world, with increased incidence in developing countries like India, China, Bangladesh, etc. Shigella spp. is diarrheal pathogen, producing mild to acute diarrhoea specifically in infants and children below 5 years of age [1]. Recent reports on global disease burden of Shigellosis, have evaluated Shigella as the second leading cause of mortality associated with diarrhoea across all ages, whereas Enterotoxigenic Escherichia coli (ETEC) was the eighth leading cause of diarrheal deaths [2]. Khalil et al. (2018) found a total of 212,438 deaths due to shigellosis worldwide which accounted for 13.2% of all diarrheal deaths all over the world (Figure 1). Shigella was also responsible for 63,713 deaths among children younger than 5 years [2]. Besides, studies have indicated an association of shigellosis with reduced linear growth and poor weight gain in children aged 2–5 years, thus contributing to global stunting [3]. Shigellosis is predominant in children having minimal access to clean water, living in poor hygiene and sanitation conditions and lacking proper nutrition.
Identification of Shigella spp. dates back to 1898, when a Japanese physician and bacteriologist Dr. Kiyoshi Shiga first isolated and characterized Shigella species [4]. The genus Shigella belongs to the family Enterobacteriaceae, with their closest relative being Escherichia coli. Shigella spp. are Gram negative, rod-shaped, non-spore forming, non-flagellated, facultative anaerobes. Shigella spp. are lysine decarboxylase negative, ornithine decarboxylase negative, oxidase negative, urease negative, catalase positive, methyl red positive and Voges Proskauer negative [5]. Shigella ferments glucose but unlike Escherichia coli, it does not ferment lactose and does not produce H2S like Salmonella. Shigella invades the colonic epithelium causing inflammation and ulceration of the large intestine, producing diarrheal symptoms varying from mild watery diarrhoea to severe dysentery characterized by blood stained stools, abdominal cramps and elevated temperature. Normally 10-100 individuals of Shigella are enough to produce the disease, thus conferring the pathogen with a very low infectious dose [6]. With a prevalence of 165 million cases worldwide and an annual death penalty of 100,000 Shigella spp. is a major scourge to public health.
On the basis of serological characteristics, the genus Shigella can be grouped into four species viz. Shigella dysenteriae, Shigella flexneri, Shigella boydii and Shigella sonnei. The ‘O’ antigen of lipopolysaccharide layer, further divides each species into multiple serotypes viz. Shigella dysenteriae has 15 serotypes, Shigella flexneri has 6 serotypes with 15 subtypes, Shigella boydii with 18 serotypes and Shigella sonnei with only one serotype [7]. The highly immunogenic ‘O’ antigen comprising of many repeats of an Oligosaccharide unit (O), exhibits widespread diversity in its biochemistry (sugar composition), number of repeats, arrangement and linkages thus accounts for the extensive diversity of Shigella spp. Of the four species, Shigella flexneri is the causative agent of 60% of the infections occurring globally and it has been studied in great depth, enhancing our perception of Shigella pathogenesis and the hidden host-pathogen intercommunication [8].
Shigella produces high levels of protein toxins encoded by both the chromosome and the plasmid like Shigella enterotoxin 1 (ShET-1), Shigella enterotoxin 2 (ShET-2) and Shiga toxin (Stx). Shiga toxin is an exotoxin only produced by Shigella dysenteriae serotype 1 and exhibits an AB5 toxin structure containing a single subunit of 32 kDa and a pentameric B subunit of 7.7 kDa, which are non-covalently associated with each other [9]. The pentameric B subunit helps in the binding of the holotoxin to specific membrane glycolipid Gb3 on the surface of vascular endothelial cells [10]. On binding to the Gb3 receptor, Stx is internalized by the process of endocytosis. The internalized vesicle fuses with the early endosome, followed by their transport to the trans-golgi network and finally into the cytosol. In the cytosol, the A subunit is cleaved at a trypsin sensitive site, into a 27 kDa A1 fragment and 4 kDa A2 fragment by furin. The catalytically active A1 fragment acts as a specific N-glycosidase and cleaves a single adenine residue from the 3′ end of 28S rRNA component of eukaryotic ribosome. This depurination blocks elongation factor-1 dependent aminoacyl tRNA binding, thus inhibiting peptide formation and damages the cell by apoptosis [11]. Impaired endothelial cells in turn release cytokines which further augment the inflammatory environment, causing cell damage by altering homeostatic control and promoting interaction of inflammatory cells and endothelial cells [12].
Shigella invasion and subsequent infection is mediated by its Type III Secretion System (T3SS). The T3SS apparatus and the effectors are encoded by a ∼220 kb large plasmid present in Shigella [13]. A 31 kb entry region within the virulence plasmid codes for the structural components of T3SS molecular syringe (Figure 2), the majority of T3SS secreted effectors and key transcriptional activators (VirB, MxiE) that facilitate Shigella invasion and dissemination [14]. The synthesis and the expression of T3SS and its effectors is stringently regulated and responds to environmental cues encountered while transport through the host.
A vital trigger that brings about T3SS expression and secretion of first wave of effectors is a swap to 37°C. However, pH, osmolarity and iron availability are other factors affecting expression and secretion of virulence factors [8]. A temperature of 37°C brings about the expression of the transcriptional activator VirF and VirB. VirF in turn induces expression of the actin nucleator and adhesin IcsA, thus stimulating actin remodelling. VirB upregulates the transcription of the parts assembling T3SS i.e., the mxi and spa genes, the first round of effectors required for cellular invasion [15].
The T3SS is composed of a basal body that spans the bacterial membrane with a needle like structure projecting from the bacterial surface. The needle is made up of repeating MxiH subunits with IpaB-IpaD translocators forming a complex at the needle tip [16]. Recent studies have proposed that the interactions of T3SS effectors with side chains of MxiH's amino acids play a crucial role in the release of effectors [17].
The predominant virulence factors of Shigella are the Invasion plasmid antigens, namely, IpaA, IpaB, IpaC, IpaD, IpaH. The needle tip of T3SS is composed of a pentamer of IpaD which serves as an attachment site for further recruitment of effectors [18].
During transit through the small intestine, IpaD undergoes a bile-salt dependent conformational change which results in the attachment of the hydrophobic effector IpaB [19]. The IpaB-IpaD complex thus formed keeps the needle complex in a closed form.
The T3SS activity is strictly controlled during the infection cycle, whereby T3SS is active only during entry and cell to cell spread but remains shut during cytosolic growth [20]. T3SS is activated by direct contact of the needle tip with the cholesterol and lipid rafts of the host plasma membrane [21]. Upon encounter with the host cell membrane, IpaB goes through a conformational change and stimulates recruitment of the next effector IpaC. The resulting IpaB-IpaC conjugate creates pores in the host cell membrane thus leading to effector influx. Following successful pore formation, MxiC is secreted first which serves as a guard and blocks the secretion of first wave of effectors, thereby ensuring the earlier release of IpaB, IpaC, IpaD factors [22]. The release of MxiC now clears the way for subsequent effector secretion.
The release of IpaB and IpaC, frees their chaperone IpgC, which forms an active complex with MxiE, liberated from the inhibitory effect of OspD1 by its seepage. The MxiE-IpgC complex now elicits the secretion of second wave of effectors while simultaneously upregulating the transcription of first round of effectors [23]. The injection of Shigella effectors in host cell suppresses host cell innate immune response and prolongs survival of infected cell. Shigella effector IpaH, with E3 ubiquitin ligase activity targets several proteins for degradation by host proteasomes, thus playing a crucial role in subverting host defense mechanism [24],[25].
Shigella enters the human body through ingestion of contaminated food and water. It expresses an effective acid resistant system (glutamate decarboxylase system) which endows Shigella with the ability to bear with the highly acidic environment of the stomach [26]. After successful passage through the stomach, the pathogen reaches the intestine where invasion occurs.
In order to reach the epithelial surface, Shigella escapes multiple barrier of the host. The existing gut microbiota provides resistance to Shigella infection by a handful of direct and indirect means, like releasing of inhibitors like colicin, depriving Shigella of nutrients, pushing toxins into Shigella via direct contact or simply by enhancing production of antimicrobial peptides by the host. Shigella survives the war by altering its ‘O’-antigen chain length and releasing colicins to kill the strains of competing bacteria [4]. The intestinal epithelium is enveloped by a rich layer of mucus which the pathogen penetrates by its expression of mucinase and neuraminidase [27]. Once Shigella reaches the epithelial surface, it targets the ‘M’ cells to breach the single layer intestinal epithelium [28]. ‘M’ cells are present in between the follicle associated epithelium (FAE) overlying the mucosa associated lymphoid tissue such as Peyer's patches, cryptopatches of the small intestine and colonic patches [29]. ‘M’ cells or microfold cells lack apical microvilli, has a large basal invagination and an apical microfold structure. ‘M’ cells are used as a surveillance system of the host by sampling antigens and microbes from the intestinal lumen to the basolateral side to elicit host immune response [29]. Shigella preferentially exploits these M cells to conquer the intestinal barrier and reach the basolateral side of polarized colonocytes. The reduced levels of mucus and antimicrobial peptides make M cells an easy target for entry. It has been highlighted by some recent investigations that Shigella mainly travels in M cells within endocytic vacuoles, thus, leaving the microfold cells intact after its exit [30]. After release from M cells, Shigella is phagocytosed by the macrophages and dendritic cells strategically located in the lymphoid pocket (Figure 3).
However, recently developed Guinea pig model of Shigellosis has shown that the entry of Shigella may occur without organized lymphoid follicles [31]. Another study revealed the existence of M cells away from Peyer's patches, although no conclusive evidence could be provided to show that these distally located M cells were used by the bacteria for the purpose of entry.
Macrophages are antigen presenting cells that function to detect and phagocytose microbes and elicit an inflammatory response to mediate bacterial clearance. Shigella prefers to escape macrophages quickly and invade the colonocytes to reach their replicative niche, the epithelial cell cytosol [32]. Shigella exits from macrophages by triggering macrophage pyroptosis. Pyroptosis is an inflammatory type of cell death that serves as an antimicrobial response by releasing the intracellular pathogen into extracellular space for more efficient killing of bacteria [33]. Pyroptosis of Shigella bearing macrophages first requires the phagosomal escape of Shigella which is induced by IpaB-IpaC needle complex component of T3SS secretory pathway. IpaB-IpaC forms pores in the phagosomal membrane, leading to influx of potassium and finally rupture of phagosome with release of vacuolar contents. Once in the cytosol, the bacterial compounds can be sensed by the cytosolic pattern recognition receptors (PRRs) [34]. The 3 main PRRs viz. nucleotide binding oligomerization domain (NOD) like receptor family (NLR), Aim2 (absent in melanoma 2) like receptor family and retinoic acid inducible gene related 1 like receptor family, recognizes a great variety of MAMPS (Microbe Associated Molecular Pattern) and DAMPS (Danger Associated Molecular Patterns). Among these receptors, Aim2 and NLRC4 can directly sense bacterial double stranded DNA and conserved bacterial proteins like T3SS rod and needle proteins by NAIP (Neuronal Apoptosis Inhibitory Proteins) respectively [35]–[38]. On the other hand, receptors like NLRP1B and NLRP3 recognize MAMPS and DAMPS by their host cell changes such as production of ROS, lysosomal destabilization, etc. Activation of any of these PRRs by MAMPS and DAMPS leads to inflammasome formation and caspase-1 activation [39],[40]. Caspase-1, an aspartate specific cysteine protease, then cleaves and activates the cellular protein Gasdermin D and the proinflammatory cytokines IL-18 and IL-1β [40]. Gasdermin D creates pores in the macrophage membrane thereby releasing IL-18 and IL-1β and simultaneously leading to water influx within macrophage, macrophage swelling and ultimately rupture of the infected macrophage. In the pyroptosis of macrophage, Shigella is not functionally quiescent, but rather plays a vital part. Shigella T3SS effector IpaH acts as an E3 ubiquitin ligase and targets glomulin for proteasome degradation [25]. When present, glomulin acts as an inhibitor for NLRC4 inflammasome. Thus, glomulin degradation frees NLRC4 from its inhibitory effect, leading to inflammasome formation and macrophage death. On pyroptosis of macrophage Shigella gains access to the basolateral side of epithelium, which Shigella proceeds to invade (Figure 4).
Shigella adheres to epithelial cells by interacting with numerous host cell surface receptors like, α5β1 integrin and CD44 and promotes activation of T3SS and secretion of effectors. CD44 are present with lipid rafts at the basolateral surface of colonocytes and IpaB-CD44 binding enhances internalization of Shigella [41]. Binding of α5β1 integrin with IpaBCD complex also occurs in lipid rafts [42]. Binding within lipid rafts favours the entry of bacteria and augments its further survival in the cell. Lipid rafts are thought to prevent the fusion of bacteria holding endosomes with lysosomes, thus protecting pathogen from degradation [43]. Besides this, the unique protein and lipid composition and high cholesterol level trigger the release of bacterial effectors in vicinity of the signaling areas.
Bacteria generally employ either of the two different processes to gain access to epithelial cells:
(1) Zipper formation: this process involves intimate contact between a bacterial surface ligand and host cell membrane proteins like cadherins and integrins. This interaction stimulates the recruitment of additional host factors thus strengthening the bacteria-host cell association and local cytoskeleton remodeling at the entry site, finally enabling bacterial uptake [44]. Pathogens like Lysteria monocytogenes, Yersinia exhibits zipper type uptake into host cell.
(2) Trigger mechanism: This process involves the injection of bacterial effectors into the host cell cytoplasm triggering cytoskeleton remodeling and ruffle formation at the site of invasion, therefore allowing bacterial engulfment by micropinocytosis [45]. Actin reorganization produces cellular extensions that risen up to 10 µm above cell surface, followed by which the filopodial extensions approach towards each other, effectively capturing Shigella within a few minutes [46]. Shigella induces ruffle formation by an intricate process involving multiple effectors viz. IpaC, IpaA, VirA, IpgD, IpgB1 and IpgB2 (Table 1).
Sr No. | Invasion Proteins | Structure | Function |
1 | IpaA | Structurally IpaA is made up of a N-terminal region, a central region and a C-terminal region. The N-terminal domain constitutes the chaperone binding site, while the helical C-terminal sequence forms the binding site for vinculin. |
|
2 | IpaB | The structure of IpaB contains a N-terminal region, a central hydrophobic sequence forming two membrane-spanning domains and a C-terminal region. The N-terminal region is assumed to play a vital role in maintaining normal IpaB expression and is thought to prevent IpaB degradation in the bacterial cytoplasm by interacting with IpgC. The hydrophobic region is crucial for different functions of IpaB, while the C-terminal region may be more important for epithelial cell invasion. |
|
3 | IpaC | Structurally IpaC is composed of a N-terminal signal sequence, a chaperone binding site, a central hydrophobic region and a C-terminal binding site [50]. |
|
4 | IpaD | The structure of IpaD consists of a N-terminal chaperone binding region, a central coiled region and a C-terminal region that harbors the IpaB binding site. |
|
5 | IpgD | IpgD shares similar motifs with mammalian phosphoinositide phosphatase enzyme. |
|
6 | IpgB1, IpgB2 | IpgB1 and IpgB2 shares identical motif with guanine nucleotide exchange factors, involved in stimulating GDP-GTP exchange in Rho family of small GTPases. |
Ruffle formation and filopodium mediated capture of Shigella is followed by retraction of filopodium, which is effectively monitored by local calcium signaling in host cells [59]. Pore formation by T3SS translocators IpaB and IpaC, activates host phospholipases C-β1 and C-γ1, which catalyzes the hydrolysis of membrane PIP2, yielding IP3 and DAG. Actin reorganization at the entry site blocks diffusion of IP3 out of the cell. IP3 is thus compelled to bind to IP3 receptors localized on endoplasmic reticulum. This binding interaction brings about a conformational change in IP3 receptors, opening calcium channels and causing a localized influx of calcium ions in epithelial cells [60]. The rise in intracellular calcium levels triggers opening of connexin hemichannels in the plasma membrane, releasing ATP through it. ATP sensing then leads to Erk1/2 activation, which induces actin retrograde flow, thus causing filopodial retraction [61].
The entry of Shigella into host cells within single membrane bound vacuoles and its release into the cytosol by vacuolar lysis are rapid events occurring within a span of 15 minutes. A functional T3SS with IpaB, IpaC, IpaD were found to carry out phagosomal rupture efficiently. The insertion of IpaB-IpaC complex into vacuolar membrane by pore formation would lead to destabilization of vacuolar membrane. The passage of T3SS effectors via the pores would further interfere with the membrane integrity, thus promoting vacuolar rupture [62]. IpgD promotes vacuolar lysis by recruiting small GTPase Rab11 to macropinosomes formed during ruffle formation and pathogen entry [56]. Studies have shown the association of Rab11 containing macropinosomes with Shigella containing vacuole just prior to the rupture, indicating a role of Rab11 in triggering vacuolar lysis (Figure 5).
However, the precise mechanism by which Rab11 influences phagosomal rupture without fusing with it, is still a matter of investigation. Recent studies have revealed that phosphoinositide phosphatase activity of IpgD is required for successful recruitment of Rab11 to macropinosomes [56].
The host deploys multiple strategies to target Shigella for destruction and prevent further invasion. Before entering phagosome, Shigella is prone to recognition by the host complement system and may result in masking of the bacteria with complement fragments. Once in the host cell cytosol, these complement fragments interact with autophagy marker ATG16L1, thus selecting the cell for autophagy [63]. Shigella antagonizes this destructive process by releasing the effector IcsP, which removes the coating of complement fragments from the bacterial surface by its proteolytic activity.
Canonical and non-canonical autophagic pathways play a vital role in bacterial clearance. The membrane damage created by Shigella T3SS during phagosomal invasion, exposes the sugar-coated luminal side of the phagosome, which is recognized by two carbohydrate binding proteins, viz. Galectin-3 and Galectin-8. These carbohydrate binding proteins link the recognition of luminal side of the vacuole to the recruitment of autophagic markers, thereby stimulating autophagy. Shigella secretes the effector IcsB, which forms a complex with TocA-1 (Transducer of CDC42 dependent actin assembly 1) and prevents the recruitment of LC3 and other autophagic markers [64].
Shigella prevents autophagosome formation by the T3SS effector VirA. VirA prevents ER to Golgi vesicular trafficking by converting the GTPase Rab1 from its GTP bound active state to its inactive GDP bound form by stimulating its GTPase activity [65],[66]. Vesicular trafficking is also monitored by ARFs (ADP ribosylation factors) [67]. Shigella virulence factor IpaJ, detaches ARF1 from Golgi membrane by cleaving the myristoyl moiety from ARF1, thereby obstructing vesicular trafficking from ER to ER-Golgi intermediate compartment (ERGIC). Obstruction of ER to Golgi vesicular trafficking does another favour for Shigella by simultaneously suppressing the STING protein mediated host innate response. STING that is anchored in the ER membrane, is capable of sensing bacterial cyclic dinucleotides, which triggers STING activation and translocation to ERGIC, where it associates with TBK1 and IRF3 (transcription factor) [68]. Activated IRF3 in turn induces expression of antiviral interferon. Disruption of ER to golgi trafficking by VirA, IcsB and IpaJ hinders STING mediated interferon release. Phagosomal rupture releases Shigella into the epithelial cell cytosol, where the bacteria replicate to multiple copies and eventually disseminate into surrounding epithelial cells to enlarge its replicative niche. In the cytosol Shigella is highly receptive to identification by the cytosolic PRR and rapidly targets it for elimination. Shigella restricts recognition of MAMPs by PRRs, by altering its LPS from a hexa-acylated form, easily noticeable by TLR4 and caspase-11 to a tetra and tri-acylated form, which is less identifiable [69]. Shigella also limits caspase-4 activation by secretion of the T3SS effector OspC3. Activated Caspase-4 is a tetramer comprising of two p19 and two p10 subunits. OspC3 binds to one p19 subunit and blocks the assembly and activation of Caspase-4 tetramer [70]. Shigella spreads to the neighbouring epithelial cells by influencing host actin filament remodelling by the secretion of the effector IcsA. IcsA resembles the small GTPase CDC42 and recruits WASP protein, which in turn binds globular actins and activates Arp2-Arp3 complex to induce actin polymerization. However, this IcsA is easily targeted by the host for autophagic capture. In this regard, the host uses a specific GTP binding protein, septins, that binds to IcsA associated actin polymers and envelops the bacteria into septin cages and recruits p62 and NDP52, thus marking them for autophagy [71]. Shigella counteracts this autophagic process again using IcsB which binds IcsA, thus competitively inhibiting Atg5 binding by concealing its recognition site [72]. IcsB also helps the pathogen to escape from the phagosome, thus indirectly helping its spread to other host cells. In IcsB mutant Shigella strains, the pathogen fails to undergo phagosomal lysis thus eventually matures to autolysosome [73]. Shigella infection also results in DNA damage of the host cell, which induces the p53 mediated proapoptotic signaling. Shigella overcomes this challenge by using another effector VirA. VirA binding to calpastatin, releases calpain from its inhibitory effect. Degradation of calpastatin frees up calpain which now targets p53 for degradation [74]. Besides VirA, p53 proapoptotic pathway is also antagonized by the phosphoinositide phosphatase activity of IpgD which liberates PI5P upon action on PIP2. PI5P in turn induces activation of the EGF receptor and the subsequent PI3kinase/Akt prosurvival pathway [75]. Activated Akt in turn phosphorylates the E3 ubiquitin ligase Mdm2, which carries out p53 degradation [76]. Besides autophagy, the host tries to seize Shigella replication by employing the guanylate binding proteins (GBPs), those are induced upon γ-interferon stimulation. GBPs augment cell autonomous antimicrobial responses to intracellular pathogens, thus challenging pathogen survival and multiplication. Shigella subverts this guanylate binding proteins by tagging them for ubiquitin mediated proteasomal degradation, using IpaH9.8, a T3SS effector [77]. Shigella infection also results in transition of mitochondrial permeability, which leads to the release of cytochrome c. Sensing of cytochrome c by APAF1, brings about formation of apoptosome and subsequent activation of caspase-9 and caspase-3, ultimately culminating in apoptosis [78]. Shigella is thought to prevent this caspase-3 mediated apoptosis by use of multiple factors, viz. FimA, Spa15m and IpgD. Association of FimA with mitochondrial outer membrane protein Vpac, enhances the affinity of Vpac for hexokinase, therefore preventing hexokinase dissociation from the mitochondrial membrane [79]. This inhibits Bax translocation to the outer membrane and formation of pores by Bax-Bak complex, through which cytochrome c would have been released. IpgD releases PI5P which prevents apoptosis by stimulating Akt activation as mentioned earlier [80]. Shigella infection also results in oxidative stress of the cell which leads to cell necrosis. This destructive process is antagonized by Shigella peptidoglycan which activates the cytosolic PRR Nod-1. Nod-1 in turn activates Rip2-IKKβ-NF-κβ signaling cascade, that upregulates the expression of potent antiapoptotic factors, thereby obstructing the prodeath pathways [81].
The replication of Shigella in the epithelial cell cytosol, is accompanied by certain metabolic changes. In order to sustain in the poor oxygen conditions of the epithelial cytosol, Shigella enhances expression of glycolytic enzymes and reduces the production of enzymes involved in aerobic oxidative pathways. Shigella also upregulates expression of transporter proteins for glucose and its derivatives [82].
The epithelial cytosol is not a stressful environment as Shigella replicates with a very high growth rate in the epithelial cytosol, doubling around every 37 minutes [83]. Although the cytosol is enriched in nutrients, the pathogen does not have adequate access to some fatty acids, some amino acids like proline and particularly iron. The host captures free iron through multiple iron binding proteins to get rid of its toxicity, while simultaneously depriving the pathogen of iron. Shigella overpowers this difficulty by intensifying the production of siderophores and by capturing ferric or ferrous iron, through a handful of transporters specific for iron [83]. However, although the cytosol provides the pathogen with a significant proportion of nutrients, Shigella acquires its energy from pyruvate, a metabolite present in ample quantities in the epithelial cell [83]. Shigella oxidizes pyruvate to acetate, liberating one ATP per pyruvate molecule. The acetate pathway is used in spite of its poor energy yield because of its speedy generation of energy to sustain rapid growth. Shigella infection therefore does not interfere with host cell energy yielding process, but rather uses an alternative pathway to support its living.
Shigella infection is followed by a shutdown in host protein synthesis [84]. This is brought about by the activation of host amino acid starvation response which results in activation of the sensor kinase GCN-2 (General control non-depressible 2). GCN-2 phosphorylates Eif2α, which inhibits initiation of protein synthesis [85]. On the contrary, Eif2α stimulates translation of ATF4, a transcription factor, which along with ATF3, upregulates expression of genes related to stress response and safety against oxidative damage. The nutrient stress response blocks mTOR pathways which further prevents translation and induces autophagy. The reduction in protein synthesis and increased metabolism of amino acids supplies Shigella with excess amino acids to maintain its growth. But Shigella opposes mTOR inhibition due to the autophagic stimulation associated with it. Using the T3SS effector OspB, the pathogen activates mTOR complex1, with the help of scaffolding protein Gap1 [86].
Shigella manages to preserve its cytosolic niche by encouraging the adhesion of infected epithelial cells to the basal membrane and blocking cell division. Shigella takes help of the T3SS effector OspE which binds to focal adhesion and forms OspE-ILK (Integrin like kinase) complex that works to prevent focal adhesion dismantling [87],[88]. Shigella blocks cell division using IpaB. IpaB interferes with Mad2l2 and inhibits it from binding Cdh2, thus making Cdh2 constitutively active [89]. Cdh2 stops cells from undergoing mitosis by suppressing mitotic cyclins needed to progress into mitosis. Besides, PI5P liberated by IpgD action on PIP2, promotes the internalization and degradation of ICAM-1 in infected cells, thereby reducing neutrophil recruitment and subsequent bacterial clearance [90].
Inflammation of the colonic epithelium favours Shigella invasion in the early stages of infection. However, Shigella undertakes numerous strategies to demolish host inflammatory response. The host employs, Toll-like receptors, which are integral membrane proteins to carry out recognition of MAMPS and DAMPS in the extracellular environment while PRRs mediate intracellular sensing of MAMPs and DAMPs. Recognition by different TLRs and PRRs elicits a wide range of host responses. However, a single MAMP can be sensed by multiple receptors, activating multiple response pathways [91]. Sensing of Shigella peptidoglycan by the Nod-1 PRR, during pathogen invasion activates NF-κB pathway in a Rip2 dependent manner [92],[93]. During cytosolic growth of Shigella, the bacterial metabolite heptose-1,7-bisphosphate is sensed by the host TIFA protein which also stimulates NF-κB pathway, via TRAF6 activation [94]. The host cells can also perceive the rupture of phagosome membrane and activates protein kinase C, which further activates NF-κB pathway in a TRAF2 dependent manner. NF-κB, which is present in inactive state in the cytoplasm due to the inhibitory effect of IκBα, is now freed by the phosphorylation and proteasomal degradation of IκBα by IKK. Active NF-κB now moves into the nucleus, thus promoting transcription of target genes. Shigella opposes the NF-κB pathway using nine effectors, which work to inhibit IKK activation and prevent NF-κB binding to the promoter region of target genes, thus downregulating target gene expression. The activation of NF-κB pathway via TRAF6 is antagonized via the effector OspI. OspI binds to UBC13 which carries out ubiquitination and activation of TRAF6 [95]. Deamination of UBC13 at Gln-100 position by OspI, impairs its E2 activity, thus hampering TRAF6 activation [96]. Shigella counteracts TRAF2 activation with the effectors IpaH0722, IpaH1.4 and IpaH2.5. IpaH0722 carries out proteasomal degradation of TRAF2, IpaH1.4 and IpaH2.5 targets HOIP (a protein that carries out activation of NF-κB downstream of TRAF) for proteasomal degradation [97]. Shigella also subverts NF-κB pathway using the effector OspF. OspF induces chromatin condensation and makes them transcriptionally inactive by regulating phosphorylation of histone H3 and heterochromatin protein 1γ of the proinflammatory target genes [98]. During pathogen invasion, sensing of bacterial LPS by TLR4 in the extracellular environment and recognition of bacterial peptidoglycan composed of diaminopimelic acid by the cytosolic PRR NOD1 activates the MAPK pathway. MAPK pathway involves the phosphorylation and activation of a cascade of MAPKs, viz. ERK, JNK, p38, finally culminating in increased expression of transcription factors. The pathogen counteracts MAPK activation with the effector OspF [99]. OspF inhibits expression of MAPK induced proinflammatory genes by deactivating Erk1/2 and p38, by irreversibly dephosphorylating threonine residues of the above-mentioned MAPKs [100]. The host uses connexin hemichannels to allow exchange of ATP between neighbouring cells and to release ATP into the extracellular environment. Abrupt speedy release of ATP into the extracellular environment is perceived by the host and leads to increased expression of ATP sensing receptors. ATP sensing then leads to a diverse range of host responses, viz. phagocytosis, NF-κB signaling, rise in the levels of proinflammatory cytokines and formation of inflammasome. Shigella wins the contest with the help of the effector IpgD. IpgD, as mentioned earlier, upon acting on PIP2 releases PI5P, that effectively plugs the connexin channel, thereby hindering ATP movement [101]. Therefore, Shigella uses myriads of effectors, which interferes with multiple host cell pathways and crushes host inflammatory response, ensuring its survival and replication in the epithelial cell cytosol.
Despite the major studies in the experimental lab aimed towards the discovery of Shigella inhibitors/vaccines, till date no major success stories have been made available. This is exactly where computational investigations, both aimed towards genome and proteome analysis as well as virtual screening based drug discovery programs need to be embraced internationally to identify suitable targets as well as candidate small molecules from natural and synthetic sources, which have the potential to eradicate the threat of Shigella pathogenesis and reduce disease incidences across the globe. Few in silico reports have come up in recent years to accelerate the process of recognition of potential drug targets. A study conducted by Morteza Hadizadeh et al. (2017) used bioinformatic analysis to identify putative drug targets in the vital pathogens of Enterobacteriaceae family [102]. Using homology-based methods and several prediction tools, a total of 35 proteins were predicted as potential drug targets. These 35 proteins were found to be unique to the pathogen, such that any drug against these targets would not produce any harmful side effects in the host. However, the novel drug targets required further evaluation before new antimicrobials are designed against them. As the T3SS effectors play a pivotal role in Shigella invasion and its spread in host, new drugs can be formulated against them to prevent the pathogen. Ganguli and Chakrabarti (2011) have reported the presence of intrinsically unstructured region in the various invasion proteins of Shigella. In their work, they distinctly characterized the amino acid sequence of the proteins and identified the intrinsically unstructured region of each [103]. This data has the potential to aid future next generation drug design studies where these specific regions can be targeted for testing the efficiency of binding of newly designed drugs to these targets. An alternative approach could be the use of Comparative Genomics for the identification of potential drug targets in Shigella, by functionally annotating the Shigella hypothetical proteins. The hypothetical proteins could later be assessed for their importance in infection development and those found crucial can be considered as candidates for future drug designing. Gazi et al. functionally predicted a few hypothetical proteins from Shigella flexneri and statistically evaluated the performance of various computational tools [104]. Thus, it is evident that computational studies can significantly reduce the time of drug discovery and simultaneously bring down the wastage of biological resources and expenditure.
Despite the advances in the understanding of the role of Shigella invasion proteins in the pathogenesis cascade, structural investigations have been limited. The following pathway has been utilized for generation of theoretical models (Figure 6).
The review has revealed in detail, how Shigella influences its uptake into host cells and prolongs its survival in the host by subverting both the host innate and adaptive immune responses. Shigella overcomes the gut microbiome and mucus layer to reach the intestinal epithelium. Until now, only little is known about the role of gut microbiota in preventing Shigella infection and the exact interactions between them. The resident microbiome may interfere with Shigella survival by depriving them of nutrients, space or may facilitate their clearance by stimulating the release of host antimicrobial peptides. Studies have shown that treatment of the host with antimicrobials like streptomycin, brings down the population of gut microbes, indirectly favoring Shigella infection [15],[105]. Similar study conducted by Medeiros et al. have shown increased susceptibility of mice to shigellosis, that are previously treated with antibiotic cocktails, suggesting a preventive role of gut microbiome in enteric infections [106]. Presence of Prevotella species among the gut commensals was also found to downregulate Shigella infection in one macaque genotype, thus necessitating further investigation. In the era of increased antimicrobial resistance, where it is quite complicated to come up with a suitable drug to fight shigellosis, investigations on interactions of Shigella with the gut microbiome may open up new treatment avenues.
[1] |
Kotloff KL, Nataro JP, Blackwelder WC, et al. (2013) Burden and aetiology of diarrhoeal disease in infants and young children in developing countries (the Global Enteric Multicenter Study, GEMS): A prospective, case-control study. Lancet 382: 209-222. doi: 10.1016/S0140-6736(13)60844-2
![]() |
[2] |
Khalil IA, Troeger C, Blacker BF, et al. (2018) Morbidity and mortality due to shigella and enterotoxigenic Escherichia coli diarrhoea: The Global Burden of Disease Study 1990–2016. Lancet Infect Dis 18: 1229-1240. doi: 10.1016/S1473-3099(18)30475-4
![]() |
[3] |
Rogawski ET, Liu J, Platts-Mills JA, et al. (2018) Use of quantitative molecular diagnostic methods to investigate the effect of enteropathogen infections on linear growth in children in low-resource settings: longitudinal analysis of results from the MAL-ED cohort study. Lancet Glob Health 6: e1319-e1328. doi: 10.1016/S2214-109X(18)30351-6
![]() |
[4] |
Trofa AF, Ueno-Olsen H, Oiwa R, et al. (1999) Dr. Kiyoshi Shiga: discoverer of the dysentery bacillus. Clin Infect Dis 29: 1303-1306. doi: 10.1086/313437
![]() |
[5] | van der Ploeg CA, Vinas MR, Terragno R, et al. (2010) Laboratory protocol: “Serotyping of Shigella spp”.1-24. |
[6] |
Kotloff KL, Riddle MS, Platts-Mills JA, et al. (2018) Shigellosis. Lancet 391: 801-812. doi: 10.1016/S0140-6736(17)33296-8
![]() |
[7] |
Muthuirulandi Sethuvel DP, Devanga Ragupathi NK, Anandan S, et al. (2017) Update on: Shigella new serogroups/serotypes and their antimicrobial resistance. Lett Appl Microbiol 64: 8-18. doi: 10.1111/lam.12690
![]() |
[8] |
Schroeder GN, Hilbi H (2008) Molecular pathogenesis of Shigella spp.: controlling host cell signaling, invasion, and death by type III secretion. Clin Microbiol Rev 21: 134-156. doi: 10.1128/CMR.00032-07
![]() |
[9] |
Pacheco AR, Sperandio V (2012) Shiga toxin in enterohemorrhagic E. coli: regulation and novel anti-virulence strategies. Front Cell Infect Microbiol 2: 81. doi: 10.3389/fcimb.2012.00081
![]() |
[10] |
Jacewicz M, Jacewicz M, Clausen H, et al. (1986) Pathogenesis of Shigella Diarrhea. XI. Isolation of a Shigella Toxin-Binding Glycolipid from Rabbit Jejunum and HeLa Cells and Its Identification as Globotriaosylceramide. J Exp Med 163: 1391-1404. doi: 10.1084/jem.163.6.1391
![]() |
[11] |
Lee MS, Cherla RP, Tesh VL (2010) Shiga toxins: intracellular trafficking to the ER leading to activation of host cell stress responses. Toxins 2: 1515-1535. doi: 10.3390/toxins2061515
![]() |
[12] |
Cotran RS, Pober JS (1989) Effects of Cytokines on Vascular Endothelium: Their Role in Vascular and Immune Injury. Kidney Int 35: 969-975. doi: 10.1038/ki.1989.80
![]() |
[13] |
Parsot C (2009) Shigella type III secretion effectors: how, where, when, for what purposes? Curr Opin Microbiol 12: 110-116. doi: 10.1016/j.mib.2008.12.002
![]() |
[14] |
Mattock E, Blocker AJ (2017) How do the virulence factors of Shigella work together to cause disease? Front Cell Infect Microbiol 7: 64. doi: 10.3389/fcimb.2017.00064
![]() |
[15] | Schnupf P, Sansonetti PJ (2019) Shigella Pathogenesis: New Insights through Advanced Methodologies. Microbiol Spectr 7. |
[16] |
Demers J-P, Habenstein B, Loquet A, et al. (2014) High-resolution structure of the Shigella type-III secretion needle by solid-state NMR and cryo-electron microscopy. Nat Commun 5: 4976. doi: 10.1038/ncomms5976
![]() |
[17] |
Dohlich K, Zumsteg AB, Goosmann C, et al. (2014) A substrate fusion protein is trapped inside the type III secretion system channel in Shigella flexneri. PLoS Pathog 10: e1003881. doi: 10.1371/journal.ppat.1003881
![]() |
[18] |
Epler CR, Dickenson NE, Bullitt E, et al. (2012) Ultrastructural analysis of IpaD at the tip of the nascent MxiH typeIII secretion apparatus of Shigella flexneri. J Mol Biol 420: 29-39. doi: 10.1016/j.jmb.2012.03.025
![]() |
[19] |
Barta ML, Guragain M, Adam P, et al. (2012) Identification of the bile salt binding site on IpaD from Shigella flexneri and the influence of ligand binding on IpaD structure. Proteins 80: 935-945. doi: 10.1002/prot.23251
![]() |
[20] |
van der Goot FG, Tran Van Nhieu G, Allaoui A, et al. (2004) Rafts can trigger contact-mediated secretion of bacterial effectors via a lipid-based mechanism. J Biol Chem 279: 47792-47798. doi: 10.1074/jbc.M406824200
![]() |
[21] |
Hayward RD, Cain RJ, McGhie EJ, et al. (2005) Cholesterol binding by the bacterial type III translocon is essential for virulence effector delivery into mammalian cells. Mol Microbiol 56: 590-603. doi: 10.1111/j.1365-2958.2005.04568.x
![]() |
[22] |
Roehrich AD, Bordignon E, Mode S, et al. (2017) Steps for Shigella gatekeeper protein MxiC function in hierarchical type III secretion regulation. J Biol Chem 292: 1705-1723. doi: 10.1074/jbc.M116.746826
![]() |
[23] |
Parsot C, Ageron E, Penno C, et al. (2005) A secreted anti-activator, OspD1, and its chaperone, Spa15, are involved in the control of transcription by the type III secretion apparatus activity in Shigella flexneri. Mol Microbiol 56: 1627-1635. doi: 10.1111/j.1365-2958.2005.04645.x
![]() |
[24] |
Rohde JR, Breitkreutz A, Chenal A, et al. (2007) TypeIII secretion effectors of the IpaH family are E3 ubiquitin ligases. Cell Host Microbe 1: 77-83. doi: 10.1016/j.chom.2007.02.002
![]() |
[25] |
Ashida H, Sasakawa C (2017) Bacterial E3 ligase effectors exploit host ubiquitin systems. Curr Opin Microbiol 35: 16-22. doi: 10.1016/j.mib.2016.11.001
![]() |
[26] |
Yang G, Wang L, Wang Y, et al. (2015) hfq regulates acid tolerance and virulence by responding to acid stress in Shigella flexneri. Res Microbiol 166: 476-485. doi: 10.1016/j.resmic.2015.06.007
![]() |
[27] | Haider K, Hossain A, Wanke C, et al. (1993) Production of Mucinase and Neuraminidase and Binding of Shigella to Intestinal Mucin. J Diarrheal Dis Res 11: 88-92. |
[28] |
Sansonetti PJ, Arondel J, Fontaine A, et al. (1991) OmpB (osmo-regulation) and icsA (cell-to-cell spread) mutants of Shigella flexneri: vaccine candidates and probes to study the pathogenesis of shigellosis. Vaccine 9: 416-422. doi: 10.1016/0264-410X(91)90128-S
![]() |
[29] |
Miller H, Zhang J, Kuolee R, et al. (2007) Intestinal M cells: the fallible sentinels? World J Gastroenterol 13: 1477-1486. doi: 10.3748/wjg.v13.i10.1477
![]() |
[30] |
Sansonetti PJ, Arondel J, Cantey JR, et al. (1996) Infection of rabbit Peyer's patches by Shigella flexneri: effect of adhesive or invasive bacterial phenotypes on follicle-associated epithelium. Infect Immun 64: 2752-2764. doi: 10.1128/IAI.64.7.2752-2764.1996
![]() |
[31] |
Shim DH, Suzuki T, Chang SY, et al. (2007) Newanimal model of shigellosis in the guinea pig: its usefulness for protective efficacy studies. J Immunol 178: 2476-2482. doi: 10.4049/jimmunol.178.4.2476
![]() |
[32] |
Ashida H, Kim M, Sasakawa C (2014) Manipulation of the host cell death pathway by Shigella. Cell Microbiol 16: 1757-1766. doi: 10.1111/cmi.12367
![]() |
[33] |
Jorgensen I, Miao EA (2015) Pyroptotic cell death defends against intracellular pathogens. Immunol Rev 265: 130-142. doi: 10.1111/imr.12287
![]() |
[34] |
Storek KM, Monack DM (2015) Bacterial recognition pathways that lead to inflammasome activation. Immunol Rev 265: 112-129. doi: 10.1111/imr.12289
![]() |
[35] |
Suzuki S, Franchi L, He Y, et al. (2014) Shigella type III secretion protein MxiI is recognized by Naip2 to induce Nlrc4 inflammasome activation independently of Pkcδ. PLoS Pathog 10: e1003926. doi: 10.1371/journal.ppat.1003926
![]() |
[36] |
Miao EA, Mao DP, Yudkovsky N, et al. (2010) Innate immune detection of the type III secretion apparatus through the NLRC4 inflammasome. Proc Natl Acad Sci USA 107: 3076-3080. doi: 10.1073/pnas.0913087107
![]() |
[37] |
Yang J, Zhao Y, Shi J, et al. (2013) Human NAIP and mouse NAIP1 recognize bacterial type III secretion needle protein for inflammasome activation. Proc Natl Acad Sci USA 110: 14408-14413. doi: 10.1073/pnas.1306376110
![]() |
[38] |
Rayamajhi M, Zak DE, Chavarria-Smith J, et al. (2013) Cutting edge: mouse NAIP1 detects the type III secretion system needle protein. J Immunol 191: 3986-3989. doi: 10.4049/jimmunol.1301549
![]() |
[39] |
Jorgensen I, Miao EA (2015) Pyroptotic cell death defends against intracellular pathogens. Immunol Rev 265: 130-142. doi: 10.1111/imr.12287
![]() |
[40] |
Shi J, Gao W, Shao F (2017) Pyroptosis: gasdermin-mediated programmed necrotic cell death. Trends Biochem Sci 42: 245-254. doi: 10.1016/j.tibs.2016.10.004
![]() |
[41] |
Skoudy A, Mounier J, Aruffo A, et al. (2000) CD44 binds to the Shigella IpaB protein and participates in bacterial invasion of epithelial cells. Cell Microbiol 2: 19-33. doi: 10.1046/j.1462-5822.2000.00028.x
![]() |
[42] |
Watarai M, Funato S, Sasakawa C (1996) Interaction of Ipa proteins of Shigella flexneri with α5β1 integrin promotes entry of the bacteria into mammalian cells. J Exp Med 183: 991-999. doi: 10.1084/jem.183.3.991
![]() |
[43] |
Helms JB, Zurzolo C (2004) Lipids as targeting signals: lipid rafts and intracellular trafficking. Traffic 5: 247-254. doi: 10.1111/j.1600-0854.2004.0181.x
![]() |
[44] |
Tran Van Nhieu G, Isberg RR (1993) Bacterial internalization mediated by beta 1 chain integrins is determined by ligand affinity and receptor density. EMBO J 12: 1887-1895. doi: 10.1002/j.1460-2075.1993.tb05837.x
![]() |
[45] |
Sansonetti PJ, Tran Van Nhieu G, Egile C (1999) Rupture of the intestinal epithelial barrier and mucosal invasion by Shigella flexneri. Clin Infect Dis 28: 466-475. doi: 10.1086/515150
![]() |
[46] |
Francis CL, Ryan TA, Jones BD, et al. (1993) Ruffles Induced by Salmonella and Other Stimuli Direct Macropinocytosis of Bacteria. Nature 364: 639-642. doi: 10.1038/364639a0
![]() |
[47] |
Tran Van Nhieu G, Ben-Ze'ev A, Sansonetti PJ (1997) Modulation of bacterial entry into epithelial cells by association between vinculin and the Shigella IpaA invasin. EMBO J 16: 2717-2729. doi: 10.1093/emboj/16.10.2717
![]() |
[48] |
Izard T, Tran Van Nhieu G, Bois PRJ (2006) Shigella applies molecular mimicry to subvert vinculin and invade host cells. J Cell Biol 175: 465-475. doi: 10.1083/jcb.200605091
![]() |
[49] |
Bourdet-Sicard R, Rudiger M, Jockusch BM, et al. (1999) Binding of the Shigella protein IpaA to vinculin induces F-actin depolymerization. EMBO J 18: 5853-5862. doi: 10.1093/emboj/18.21.5853
![]() |
[50] |
Terry CM, Picking WL, Birket SE, et al. (2008) The C-terminus of IpaC is required for effector activities related to Shigella invasion of host cells. Microb Pathog 45: 282-289. doi: 10.1016/j.micpath.2008.06.003
![]() |
[51] | Russo BC, Stamm LM, Raaben M, et al. (2016) Intermediate filaments enable pathogen docking to trigger type 3 effector translocation. Nat Microbiol 7: 1-24. |
[52] |
Tran Van Nhieu G, Caron E, Hall A, et al. (1999) IpaC induces actin polymerization and filopodia formation during Shigella entry into epithelial cells. EMBO J 18: 3249-3262. doi: 10.1093/emboj/18.12.3249
![]() |
[53] |
Mounier J, Popoff MR, Enninga J, et al. (2009) The IpaC carboxyterminal effector domain mediates Src-dependent actin polymerization during Shigella invasion of epithelial cells. PLoS Pathog 5: e1000271. doi: 10.1371/journal.ppat.1000271
![]() |
[54] |
Shaikh NM, Terajima J, Watanabe H (2003) IpaC of Shigella binds to the C-terminal domain of β-catenin. Microb Pathog 35: 107-117. doi: 10.1016/S0882-4010(03)00093-7
![]() |
[55] |
Niebuhr K, Giuriato S, Pedron T, et al. (2002) Conversion of PtdIns(4,5)P2 into PtdIns(5)P by the S. flexneri effector IpgD reorganizes host cell morphology. EMBO J 21: 5069-5078. doi: 10.1093/emboj/cdf522
![]() |
[56] |
Weiner A, Mellouk N, Lopez-Montero N, et al. (2016) Macropinosomes are key players in early Shigella invasion and vacuolar escape in epithelial cells. PLoS Pathog 5: e1005602. doi: 10.1371/journal.ppat.1005602
![]() |
[57] |
Huang Z, Sutton SE, Wallenfang AJ, et al. (2009) Structural insights into host GTPase isoform selection by a family of bacterial GEF mimics. Nat Struct Mol Biol 16: 853-860. doi: 10.1038/nsmb.1647
![]() |
[58] |
Klink BU, Barden S, Heidler TV, et al. (2010) Structure of Shigella IpgB2 in complex with human RhoA. J Biol Chem 285: 17197-17208. doi: 10.1074/jbc.M110.107953
![]() |
[59] |
Bonnet M, Tran Van Nhieu G (2016) How Shigella utilizes Ca (2+) jagged edge signals during invasion of epithelial cells. Front Cell Infect Microbiol 6: 16. doi: 10.3389/fcimb.2016.00016
![]() |
[60] |
Tran Van Nhieu G, Kai Liu B, Zhang J, et al. (2013) Actin based confinement of calcium responses during Shigella invasion. Nat Commun 4: 1567. doi: 10.1038/ncomms2561
![]() |
[61] |
Romero S, Grompone G, Carayol N, et al. (2011) ATP-mediated Erk1/2activation stimulates bacterial capture by filopodia, which precedes Shigella invasion of epithelial cells. Cell Host Microbe 9: 508-519. doi: 10.1016/j.chom.2011.05.005
![]() |
[62] |
Mellouk N, Weiner A, Aulner N, et al. (2014) Shigella subverts the host recycling compartment to rupture its vacuole. Cell Host Microbe 16: 517-530. doi: 10.1016/j.chom.2014.09.005
![]() |
[63] |
Sorbara MT, Foerster EG, Tsalikis J, et al. (2018) Complement C3 drives autophagy dependent restriction of cyto-invasive bacteria. Cell Host Microbe 23: 644-652. doi: 10.1016/j.chom.2018.04.008
![]() |
[64] |
Baxt LA, Goldberg MB (2014) Host and bacterial proteins that repress recruitment of LC3 to Shigella early during infection. PLoS One 9: e94653. doi: 10.1371/journal.pone.0094653
![]() |
[65] |
Huang J, Brumell JH (2014) Bacteria-autophagy interplay: a battle for survival. Nat Rev Microbiol 12: 101-114. doi: 10.1038/nrmicro3160
![]() |
[66] |
Dong N, Zhu Y, Lu Q, et al. (2012) Structurally distinct bacterial TBC-like GAPs link Arf GTPase to Rab1 inactivation to counteract host defenses. Cell 150: 1029-1041. doi: 10.1016/j.cell.2012.06.050
![]() |
[67] |
Liu W, Zhou Y, Peng T, et al. (2018) Nε-fatty acylation of multiple membrane associated proteins by Shigella IcsB effector to modulate host function. Nat Microbiol 3: 996-1009. doi: 10.1038/s41564-018-0215-6
![]() |
[68] |
Dobbs N, Burnaevskiy N, Chen D, et al. (2015) STING activation by translocation from the ER is associated with infection and autoinflammatory disease. Cell Host Microbe 18: 157-168. doi: 10.1016/j.chom.2015.07.001
![]() |
[69] |
Paciello I, Silipo A, Lembo-Fazio L, et al. (2013) Intracellular Shigella remodels its LPS to dampen the innate immune recognition and evade inflammasome activation. Proc Natl Acad Sci USA 110: E4345-E4354. doi: 10.1073/pnas.1303641110
![]() |
[70] |
Kobayashi T, Ogawa M, Sanada T, et al. (2013) The Shigella OspC3 effector inhibits caspase-4, antagonizes inflammatory cell death, and promotes epithelial infection. Cell Host Microbe 13: 570-583. doi: 10.1016/j.chom.2013.04.012
![]() |
[71] |
Torraca V, Mostowy S (2016) Septins and bacterial infection. Front Cell Dev Biol 4: 127. doi: 10.3389/fcell.2016.00127
![]() |
[72] |
Ogawa M, Yoshimori T, Suzuki T, et al. (2005) Escape of intracellular Shigella from autophagy. Science 307: 727-731. doi: 10.1126/science.1106036
![]() |
[73] | Campbell-Valois F-X, Sachse M, Sansonetti PJ, et al. (2015) Escape of actively secreting Shigella flexneri from ATG8/LC3-positive vacuoles formed during cell-to-cell spread is facilitated by IcsB and VirA. mBio 6: e02567-14. |
[74] |
Bergounioux J, Elisee R, Prunier A-L, et al. (2012) Calpain activation by the Shigella flexneri effector VirA regulates key steps in the formation and life of the bacterium's epithelial niche. Cell Host Microbe 11: 240-252. doi: 10.1016/j.chom.2012.01.013
![]() |
[75] |
Mayo LD, Donner DB (2001) A phosphatidylinositol 3-kinase/Akt pathway promotes translocation of Mdm2 from the cytoplasm to the nucleus. Proc Natl Acad Sci USA 98: 11598-11603. doi: 10.1073/pnas.181181198
![]() |
[76] |
Ramel D, Lagarrigue F, Pons V, et al. (2011) Shigella flexneri infection generates the lipid PI5P to alter endocytosis and prevent termination of EGFR signaling. Sci Signal 4: ra61. doi: 10.1126/scisignal.2001619
![]() |
[77] | Piro A S, Hernandez D, Luoma S, et al. (2017) Detection of cytosolic Shigella flexneri via a C-terminal triple-arginine motif of GBP1inhibits actin-based motility. mBio 8: e01979-17. |
[78] |
Bhola PD, Letai A (2016) Mitochondria—judges and executioners of cell death sentences. Mol Cell 61: 695-704. doi: 10.1016/j.molcel.2016.02.019
![]() |
[79] |
Sukumaran SK, Fu NY, Tin CB, et al. (2010) A soluble form of the pilus protein FimA targets the VDAC-hexokinase complex at mitochondria to suppress host cell apoptosis. Mol Cell 37: 768-783. doi: 10.1016/j.molcel.2010.02.015
![]() |
[80] |
Pendaries C, Tronchère H, Arbibe L, et al. (2006) PtdIns5P activates the host cell PI3-kinase/Akt pathway during Shigella flexneri infection. EMBO J 25: 1024-1034. doi: 10.1038/sj.emboj.7601001
![]() |
[81] |
Carneiro LAM, Travassos LH, Soares F, et al. (2009) Shigella induces mitochondrial dysfunction and cell death in nonmyleoid cells. Cell Host Microbe 5: 123-136. doi: 10.1016/j.chom.2008.12.011
![]() |
[82] |
Pieper R, Fisher CR, Suh MJ, et al. (2013) Analysis of the proteome of intracellular Shigella flexneri reveals pathways important for intracellular growth. Infect Immun 81: 4635-4648. doi: 10.1128/IAI.00975-13
![]() |
[83] |
Kentner D, Martano G, Callon M, et al. (2014) Shigella reroutes host cell central metabolism to obtain high-flux nutrient supply for vigorous intracellular growth. Proc Natl Acad Sci USA 111: 9929-9934. doi: 10.1073/pnas.1406694111
![]() |
[84] |
Vonaesch P, Campbell-Valois F-X, Dufour A, et al. (2016) Shigella flexneri modulates stress granule composition and inhibits stress granule aggregation. Cell Microbiol 18: 982-997. doi: 10.1111/cmi.12561
![]() |
[85] |
Tattoli I, Sorbara MT, Vuckovic D, et al. (2012) Amino acid starvation induced by invasive bacterial pathogens triggers an innate host defense program. Cell Host Microbe 11: 563-575. doi: 10.1016/j.chom.2012.04.012
![]() |
[86] |
Lu R, Herrera BB, Eshleman HD, et al. (2015) Shigella effector OspB activates mTORC1 in a manner that depends on IQGAP1 and promotes cell proliferation. PLoS Pathog 11: e1005200. doi: 10.1371/journal.ppat.1005200
![]() |
[87] |
Kim M, Ogawa M, Fujita Y, et al. (2009) Bacteria hijack integrin linked kinase to stabilize focal adhesions and block cell detachment. Nature 459: 578-582. doi: 10.1038/nature07952
![]() |
[88] |
Miura M, Terajima J, Izumiya H, et al. (2006) OspE2 of Shigella sonnei is required for the maintenance of cell architecture of bacterium-infected cells. Infect Immun 74: 2587-2595. doi: 10.1128/IAI.74.5.2587-2595.2006
![]() |
[89] |
Iwai H, Kim M, Yoshikawa Y, et al. (2007) A bacterial effector targets Mad2L2, an APC inhibitor, to modulate host cell cycling. Cell 130: 611-623. doi: 10.1016/j.cell.2007.06.043
![]() |
[90] |
Boal F, Puhar A, Xuereb J-M, et al. (2016) PI5P triggers ICAM-1 degradation in Shigella infected cells, thus dampening immune cell recruitment. Cell Rep 14: 750-759. doi: 10.1016/j.celrep.2015.12.079
![]() |
[91] |
Yu S, Gao N (2015) Compartmentalizing intestinal epithelial cell toll like receptors for immune surveillance. Cell Mol Life Sci 72: 3343-3353. doi: 10.1007/s00018-015-1931-1
![]() |
[92] |
Girardin SE, Tournebize R, Mavris M, et al. (2001) CARD4/NOD1 mediates NF-κB and JNK activation by invasive Shigella flexneri. EMBO Rep 2: 736-742. doi: 10.1093/embo-reports/kve155
![]() |
[93] |
Killackey SA, Sorbara MT, Girardin SE (2016) Cellular aspects of Shigella pathogenesis: focus on the manipulation of host cell processes. Front Cell Infect Microbiol 6: 38. doi: 10.3389/fcimb.2016.00038
![]() |
[94] |
Gaudet RG, Guo CX, Molinaro R, et al. (2017) Innate recognition of intracellular bacterial growth is driven by the TIFA-dependent cytosolic surveillance pathway. Cell Rep 19: 1418-1430. doi: 10.1016/j.celrep.2017.04.063
![]() |
[95] |
Sanada T, Kim M, Mimuro H, et al. (2012) The Shigella flexneri effector OspI deamidates UBC13 to dampen the inflammatory response. Nature 483: 623-626. doi: 10.1038/nature10894
![]() |
[96] |
Nishide A, Kim M, Takagi K, et al. (2013) Structural basis for the recognition of Ubc13 by the Shigella flexneri effector OspI. J Mol Biol 425: 2623-2631. doi: 10.1016/j.jmb.2013.02.037
![]() |
[97] |
Ashida H, Nakano H, Sasakawa C (2013) Shigella IpaH0722 E3 ubiquitin ligase effector targets TRAF2 to inhibit PKC-NF-κB activity in invaded epithelial cells. PLoS Pathog 9: e1003409. doi: 10.1371/journal.ppat.1003409
![]() |
[98] |
Harouz H, Rachez C, Meijer BM, et al. (2014) Shigella flexneri targets the HP1γ subcode through the phosphothreonine lyase OspF. EMBO J 33: 2606-2622. doi: 10.15252/embj.201489244
![]() |
[99] |
Li H, Xu H, Zhou Y, et al. (2007) The phosphothreonine lyase activity of a bacterial type III effector family. Science 315: 1000-1003. doi: 10.1126/science.1138960
![]() |
[100] |
Arbibe L, Kim DW, Batsche E, et al. (2007) An injected bacterial effector targets chromatin access for transcription factor NF-kappaB to alter transcription of host genes involved in immune responses. Nat Immunol 8: 47-56. doi: 10.1038/ni1423
![]() |
[101] |
Puhar A, Tronchère H, Payrastre B, et al. (2013) A Shigella effector dampens inflammation by regulating epithelial release of danger signal ATP through production of the lipid mediator PtdIns5P. Immunity 39: 1121-1131. doi: 10.1016/j.immuni.2013.11.013
![]() |
[102] |
Hadizadeh M, Tabatabaiepour SN, Tabatabaiepour SZ, et al. (2018) Genome-Wide Identification of Potential Drug Target in Enterobacteriaceae Family: A Homology-Based Method. Microb Drug Resist 24: 8-17. doi: 10.1089/mdr.2016.0259
![]() |
[103] | Chakrabarti S, Ganguli S (2013) Structural Analyses of Shigella Invasion Proteins Reveals Non-Conserved; Intrinsically Unstructured Regions. Intl Lett Nat Sci 5: 52-58. |
[104] |
Gazi MA, Mahmud S, Fahim SM, et al. (2018) Functional Prediction of Hypothetical Proteins from Shigella flexneri and Validation of the Predicted Models by Using ROC Curve Analysis. Genomics Inform 16: e26. doi: 10.5808/GI.2018.16.4.e26
![]() |
[105] |
Martino MC, Rossi G, Martini I, et al. (2005) Mucosal lymphoid infiltrate dominates colonic pathological changes in murine experimental shigellosis. J Infect Dis 192: 136-148. doi: 10.1086/430740
![]() |
[106] |
Q S Medeiros PH, Ledwaba SE, Bolick DT, et al. (2019) A murine model of diarrhea, growth impairment and metabolic disturbances with Shigella flexneri infection and the role of zinc deficiency. Gut Microbes 10: 615-630. doi: 10.1080/19490976.2018.1564430
![]() |
1. | Tanuka Sen, Naresh K. Verma, YfiB: An Outer Membrane Protein Involved in the Virulence of Shigella flexneri, 2022, 10, 2076-2607, 653, 10.3390/microorganisms10030653 | |
2. | Fekade Beshah Tessema, Yilma Hunde Gonfa, Tilahun Belayneh Asfaw, Tigist Getachew Tadesse, Mesfin Getachew Tadesse, Archana Bachheti, Devi Prasad Pandey, Saikh M. Wabaidur, Kholood A. Dahlous, Ivan Širić, Pankaj Kumar, Vinod Kumar, Sami Abou Fayssal, Rakesh Kumar Bachheti, Flavonoids and Phenolic Acids from Aerial Part of Ajuga integrifolia (Buch.-Ham. Ex D. Don): Anti-Shigellosis Activity and In Silico Molecular Docking Studies, 2023, 28, 1420-3049, 1111, 10.3390/molecules28031111 | |
3. | Marco Calvigioni, Diletta Mazzantini, Francesco Celandroni, Emilia Ghelardi, Animal and In Vitro Models as Powerful Tools to Decipher the Effects of Enteric Pathogens on the Human Gut Microbiota, 2023, 12, 2076-2607, 67, 10.3390/microorganisms12010067 |
Sr No. | Invasion Proteins | Structure | Function |
1 | IpaA | Structurally IpaA is made up of a N-terminal region, a central region and a C-terminal region. The N-terminal domain constitutes the chaperone binding site, while the helical C-terminal sequence forms the binding site for vinculin. |
|
2 | IpaB | The structure of IpaB contains a N-terminal region, a central hydrophobic sequence forming two membrane-spanning domains and a C-terminal region. The N-terminal region is assumed to play a vital role in maintaining normal IpaB expression and is thought to prevent IpaB degradation in the bacterial cytoplasm by interacting with IpgC. The hydrophobic region is crucial for different functions of IpaB, while the C-terminal region may be more important for epithelial cell invasion. |
|
3 | IpaC | Structurally IpaC is composed of a N-terminal signal sequence, a chaperone binding site, a central hydrophobic region and a C-terminal binding site [50]. |
|
4 | IpaD | The structure of IpaD consists of a N-terminal chaperone binding region, a central coiled region and a C-terminal region that harbors the IpaB binding site. |
|
5 | IpgD | IpgD shares similar motifs with mammalian phosphoinositide phosphatase enzyme. |
|
6 | IpgB1, IpgB2 | IpgB1 and IpgB2 shares identical motif with guanine nucleotide exchange factors, involved in stimulating GDP-GTP exchange in Rho family of small GTPases. |
Sr No. | Invasion Proteins | Structure | Function |
1 | IpaA | Structurally IpaA is made up of a N-terminal region, a central region and a C-terminal region. The N-terminal domain constitutes the chaperone binding site, while the helical C-terminal sequence forms the binding site for vinculin. |
|
2 | IpaB | The structure of IpaB contains a N-terminal region, a central hydrophobic sequence forming two membrane-spanning domains and a C-terminal region. The N-terminal region is assumed to play a vital role in maintaining normal IpaB expression and is thought to prevent IpaB degradation in the bacterial cytoplasm by interacting with IpgC. The hydrophobic region is crucial for different functions of IpaB, while the C-terminal region may be more important for epithelial cell invasion. |
|
3 | IpaC | Structurally IpaC is composed of a N-terminal signal sequence, a chaperone binding site, a central hydrophobic region and a C-terminal binding site [50]. |
|
4 | IpaD | The structure of IpaD consists of a N-terminal chaperone binding region, a central coiled region and a C-terminal region that harbors the IpaB binding site. |
|
5 | IpgD | IpgD shares similar motifs with mammalian phosphoinositide phosphatase enzyme. |
|
6 | IpgB1, IpgB2 | IpgB1 and IpgB2 shares identical motif with guanine nucleotide exchange factors, involved in stimulating GDP-GTP exchange in Rho family of small GTPases. |