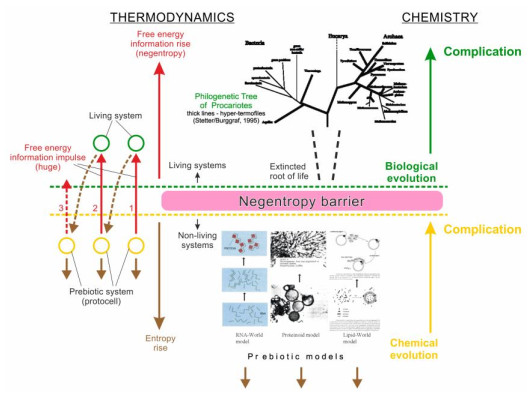
Now there is a huge variety of scenarios of prebiotic chemical evolution, culminating in the emergence of life on Earth, which demonstrates the obvious insufficiency of existing criteria for a reliable consideration of this process. This article develops the concept of thermodynamic inversion (TI concept) according to which the real succession of the formation of metabolism during the origin of life is fixed in the stages of the exit of a resting bacterial cell from anabiosis (suspended animation), just as the succession of events of phylogenesis is fixed in ontogenesis. The deepest phase of anabiosis considers by us as an intermediate state of a microorganism between non-life and life: it is no longer able to counteract the increase in entropy, but retains structural memory of the previous living state. According to the TI concept, the intermediate state between non-life and life thermodynamically corresponds to the approximate equality of the total contributions of entropy and free energy in prebiotic systems (Sc ≈ FEc). Considering such intermediate state in prebiotic systems and microorganisms as a starting point, the authors use the experimentally recorded stages of restoring the metabolic process when a resting (dormant) bacterial cell emerges from anabiosis as a guideline for identifying the sequence of metabolism origin in prebiotic systems. According to the TI concept, life originated in a pulsating updraft of hydrothermal fluid. It included four stages. 1) Self-assembly of a cluster of organic microsystems (complex liposomes). 2) Activation (formation of protocells): appearance in the microsystems a weak energy-giving process of respiration due to redox reactions; local watering in the membrane. 3) Initiation (formation of living subcells): formation of a non-enzymatic antioxidant system; dawning of the protein-synthesizing apparatus. 4) Growth (formation of living cells—progenotes): arising of the growth cell cycle; formation of the genetic apparatus.
Citation: Vladimir Kompanichenko, Galina El-Registan. Advancement of the TI concept: defining the origin-of-life stages based on the succession of a bacterial cell exit from anabiosis[J]. AIMS Geosciences, 2022, 8(3): 398-437. doi: 10.3934/geosci.2022023
[1] | Matteo Serra, Fabio Fanari, Francesco Desogus, Paolo Valera . The fluorine in surface waters: origin, weight on human health, and defluoridation techniques. AIMS Geosciences, 2022, 8(4): 686-705. doi: 10.3934/geosci.2022038 |
[2] | Diana I Bakalova, Ekaterina E Dimitrova . Optimistic expectations and life satisfaction as antecedents of emigration attitudes among Bulgarian Millennials and Zoomers. AIMS Geosciences, 2023, 9(2): 285-310. doi: 10.3934/geosci.2023016 |
[3] | William Guo . Thermal magnetic analysis on iron ores and banded iron formations (BIFs) in the Hamersley Province: Implications of origins of magnetic minerals and iron ores. AIMS Geosciences, 2023, 9(2): 311-329. doi: 10.3934/geosci.2023017 |
[4] | José Luis Sánchez-Salas, Alejandra Aguilar Ubeda, Beatriz Flores Gómez, Oscar Daniel Máynez Navarro, Miguel Ángel Méndez Rojas, Silvia Reyna Tellez, Erick R. Bandala . Inactivation of Bacterial Spores and Vegetative Bacterial Cells by Interaction with ZnO-Fe2O3 Nanoparticles and UV Radiation. AIMS Geosciences, 2017, 3(4): 498-513. doi: 10.3934/geosci.2017.4.498 |
[5] | Karla E. Campos Díaz, José L. Álvarez Cruz, Miriam L. Lira Rodríguez, Erick R. Bandala . Coupled Inverse Fluidized Bed Bioreactor with Advanced Oxidation Processes for Treatment of Vinasse. AIMS Geosciences, 2017, 3(4): 538-551. doi: 10.3934/geosci.2017.4.538 |
[6] | Valentina Albanese . Global images vs cultural images: mixed methods to deepen territorial representations. AIMS Geosciences, 2022, 8(4): 593-608. doi: 10.3934/geosci.2022032 |
[7] | Giuseppe Borruso, Ginevra Balletto . Cities as innovation poles in the digital transition. The Italian case. AIMS Geosciences, 2024, 10(4): 918-938. doi: 10.3934/geosci.2024043 |
[8] | Firoz Ahmad, Laxmi Goparaju, Abdul Qayum . Wild life habitat suitability and conservation hotspot mapping: Remote Sensing and GIS based decision support system. AIMS Geosciences, 2018, 4(1): 66-87. doi: 10.3934/geosci.2018.1.66 |
[9] | Dell’Aversana Paolo, Bernasconi Giancarlo, Chiappa Fabio . A Global Integration Platform for Optimizing Cooperative Modeling and Simultaneous Joint Inversion of Multi-domain Geophysical Data. AIMS Geosciences, 2016, 2(1): 1-31. doi: 10.3934/geosci.2016.1.1 |
[10] | David W. Schwartzman . Life’s Critical Role in the Long-term Carbon Cycle: the Biotic Enhancement of Weathering. AIMS Geosciences, 2017, 3(2): 216-238. doi: 10.3934/geosci.2017.2.216 |
Now there is a huge variety of scenarios of prebiotic chemical evolution, culminating in the emergence of life on Earth, which demonstrates the obvious insufficiency of existing criteria for a reliable consideration of this process. This article develops the concept of thermodynamic inversion (TI concept) according to which the real succession of the formation of metabolism during the origin of life is fixed in the stages of the exit of a resting bacterial cell from anabiosis (suspended animation), just as the succession of events of phylogenesis is fixed in ontogenesis. The deepest phase of anabiosis considers by us as an intermediate state of a microorganism between non-life and life: it is no longer able to counteract the increase in entropy, but retains structural memory of the previous living state. According to the TI concept, the intermediate state between non-life and life thermodynamically corresponds to the approximate equality of the total contributions of entropy and free energy in prebiotic systems (Sc ≈ FEc). Considering such intermediate state in prebiotic systems and microorganisms as a starting point, the authors use the experimentally recorded stages of restoring the metabolic process when a resting (dormant) bacterial cell emerges from anabiosis as a guideline for identifying the sequence of metabolism origin in prebiotic systems. According to the TI concept, life originated in a pulsating updraft of hydrothermal fluid. It included four stages. 1) Self-assembly of a cluster of organic microsystems (complex liposomes). 2) Activation (formation of protocells): appearance in the microsystems a weak energy-giving process of respiration due to redox reactions; local watering in the membrane. 3) Initiation (formation of living subcells): formation of a non-enzymatic antioxidant system; dawning of the protein-synthesizing apparatus. 4) Growth (formation of living cells—progenotes): arising of the growth cell cycle; formation of the genetic apparatus.
To date, there are a large number of concepts of prebiotic chemical evolution, the final stage of which was the emergence of life on Earth. Based on these approaches, different types of prebiotic chemical models that preceded living cells have been proposed: coacervate droplets [1], proteinoid microspheres [2,3], the world of RNA [4], liposomes [5,6], the world of aromatic hydrocarbons [7], as well as various combined models. Some of them are now of historical interest. Many models are formed during spontaneous self-assembly (for example, lipid vesicles), but for models of different type other mechanisms of formation are envisaged, in particular, synthesis of organic material on mineral surfaces (some clays, apatites, etc.) [8]. Recently, complex prebiotic models consisting of two or more main types of organic molecules, for example, lipids and nucleotides, have been most widely discussed [9,10,11,12].
Despite the huge differences in the proposed chemical pathways of prebiotic evolution, scientists agree that, in general, it proceeded against the background of the processes of complication of organic molecules and microsystems (models). At its initial stage, simple organic compounds synthesized from inorganic ones. In particular, these are hydrocarbons [13], formamides [14], phospholipids [15], and other molecules. More complex compounds have also synthesized, such as amino acids and even peptides [16,17,18,19,20]. Similar organic material could have been delivered from space with meteorites [21,22]. The next stage of evolution is associated with the formation of more complex compounds from simple organic molecules and macromolecules, which are usually called prebiotic microsystems (microspheres), or "protocells". According to different authors, their composition may vary greatly. For example, after the formation of lipid vesicles in the process of self-assembly, nucleotide molecules can penetrate them by diffusion. Inside, they are able to polycondense into chains of oligomers, complicating the system [12]. The process of prebiotic complication proceeding further, up to the formation of so-called "artificial cells", provides self-replication of RNA or DNA chains and leads to the separation of microsystems into two components, like living cells. All the stages of prebiotic evolution noted above have been confirmed experimentally [10,11,23].
Some researchers believe that the next stage of evolution after the formation of "artificial cells" were progenotes [12]. Progenote is the conventional name of a group of now extinct life forms that had a more primitive organization than the simplest modern cells. They were theoretically reconstructed by C. Woese based on the analysis of the phylogenetic tree of microorganisms [24]. Progenotes were alive cells. In contrast, any types of artificial cells (models) synthesized from abiogenic material are not alive. They do not manifest two key properties of living cells populations—the ability for expansion in the environment and the ability to purposeful behavior. This means that the actual moment of life origin (i.e. the key transformation that determines the appearance of a living state in prebiotic microsystems) is "hidden" in the evolutionary gap between non-living model cells and living progenote cells.
An attempt to consider the mentioned key moment of life origin as a starting point of biological evolution was made within the framework of the TI concept (the concept of "thermodynamic inversion", or the "inversion" concept) [25,26,27,28,29,30]. What is the TI concept essence? It known that the macrostate of any natural system ultimately determined by thermodynamics. Inanimate natural systems, for instance, geologically active planets or formed chains of mountains, exist (evolve) with a tendency to a natural increase in entropy associated with the dissipation of free energy in the surroundings. This follows from the 1st and 2nd laws of thermodynamics. In contrast, biological evolution paradoxically proceeds in the thermodynamically opposite direction—with a tendency to concentrate free energy and information against the background of a relative decrease in entropy. This is especially noticeable when considering the biosphere evolution as a whole. Although entropy also increases in biological systems, the concentration of free energy and information that compensates for it grows faster. This occurs due to an energetically efficient internal restructuring, coupled with the ability to intensively and purposefully respond to external influences (i.e., to periodic stress, the strength of which does not exceed the system's ability to adapt). Within the framework of the TI concept, this difference expresses through the natural system general thermodynamic balance: "the total contribution of entropy (Sc)/the total contribution of free energy (FEc)" [26,28]. According to the theoretical study by Feistel and Ebeling [31], a small part of entropy relates with informational processes. In this regard, the above ratio supplements by one more balance: "the total contribution of informational entropy (Sic)/the total contribution of information (Ic)". Accordingly, both balances are positive in non-living systems, and negative in living developing systems. In such a thermodynamic context, living systems of different levels of organization (from organisms to the biosphere as a whole) are usually defined as antientropic, or negentropic (negentropy—negative entropy). This understanding of the thermodynamic difference between non-living and living systems is generally accepted.
However, the noted difference implies a consequence that usually falls out of the attention of scientists working in the field of the origin of life: the transition of non-living prebiotic microsystems (protocells) and their clusters into the simplest living microorganisms and their populations is possible only through thermodynamic inversion. It starts when the combined contribution of free energy and information begins to dominate over the contribution of entropy [26,28]. At this moment, there is a change of dominants in the considered thermodynamic balances. According to the TI approach, the culmination of the transition, occurring in an oscillatory mode, is achieved with approximately equal balances: Sc ≈ FEc, Sic ≈ Ic. It should be added that due to the continuous fluctuations of the balances during the transition to living systems, the absolute identity in them (Sc = FEc, Sic = Ic), which could be expressed through precise mathematical calculations, seems unattainable. That is why, to qualitatively assess the thermodynamic balance in such different types of natural systems as non-living and living, it should be used more general concepts and terms. In particular, in physical systems (for example, in heat engines), entropy can be accurately calculated using the appropriate formula. To conduct a thermodynamic comparison of non-living and living systems within the framework of the inversion concept, instead of the term "entropy", the more general term "entropy contribution" is used. At the same time, the physical meaning of the term "entropy" preserved in its qualitative version ("entropy contribution"). The same applies to the terms "free energy contribution" and "information contribution".
On the early Earth, organic prebiotic microsystems and their clusters with the near-zero thermodynamic balance described above (Sc ≈ FEc, Sic ≈ Ic) were in an intermediate state between inanimate and living. From that moment, in the course of their further evolution and the shift of the thermodynamic balance in the negentropic direction, excess (supra-entropy) free energy and information appeared in them, which ensured over time the development of metabolism and the formation of biological structures.
As noted above, there is now a huge variety of views on prebiotic chemical evolution. This fact demonstrates the insufficiency of the available orienting points for its reliable consideration. Within the framework of biological evolution, the general sequence of development of biological systems—from the simplest organisms to humans and the biosphere as a whole—is well studied on the basis of data on their ontogenesis and phylogenesis. At the same time, if the evolution of life on Earth began with the simplest forms - microorganisms (apparently, predominantly thermophilic), then in their ontogenesis (the cycle of development of microbial populations) even earlier stages of a biological system's development can be recorded, up to the moment of the emergence of life.
According to the authors, these super-early stages of the emergence of life can be reflected in the sequence of processes that manifest themselves both during the transition of microorganisms to a metabolically inert state of dormancy (anabiosis) and when they leave the dormant state, what has fixed in the ontogeny of the microbial population. At the same time, the sequence of processes during the transition of a metabolically active microbial cell to an ametabolic anabiotic resting form reflects the reverse dynamics of events in the development of the initial biological system. Resting cells formed under the unfavorable for growth conditions represent, according to the inversion concept terminology, an intermediate state between life (as they retain the structural memory of it) and "non-life" (because of their inability to resist entropy, unlike forms of life, which are functionally active). Both the cell's exit from a state of dormancy and its transition to rest begin with the impact of external factors that disrupt its balance with the environment (homeostasis)—that is, with stress changes in the environment [32,33].
Concluding the introduction, let us emphasize the fundamental similarities between prebiotic microsystems in the process of their transformation into primary living subcells (according to the inversion concept) and dormant forms of microorganisms. Both those and others are in an intermediate state between "non-life" and life. This state is reversible both for dormant forms (at the initial stage of their exit from the state of anabiosis) and for prebiotic microsystems/subcells. Based on this initial assumption, we formulate the objectives of this article in the following form.
1. To characterize the intermediate state between "non-life and life" in prebiotic microsystems (protocells) and dormant anabiotic forms of microorganisms; justify the possibility of a correlation between them. 2. To use the experimentally fixed sequence of acquisition by cells of modern bacteria of a dormant anabiotic state and exit from it as a basis for identifying the stages of metabolism origin in prebiotic microsystems (protocells) and their clusters, starting from the above described moment of emergence of life (i.e., thermodynamic inversion).
The thesis substantiated in the introduction about the need for thermodynamic inversion (TI) in the process of the origin of life is a starting point of the TI concept. In the course of this thermodynamic transformation, a transition occurred from an excess of entropy, which characterizes non-living prebiotic systems, to its deficit. In other words—to the continuous existence of "supra-entropic" ("super-entropic") free energy in living systems—microorganisms and their developing populations. Accordingly, the focus is on the very moment of the emergence of life, which connects the initial non-living and emerging living state in the same (micro) system or cluster of microsystems. This moment is considered as an intermediate link between the physicochemical properties of prebiotic microsystems that are in close proximity to the transition to a living state (acquiring catalytic activity, cooperative reactions, the ability to divide), and the "sparks" of biological properties proper in primary forms life (living subcells). Among the latter, two key biological properties should be singled out: the ability to expansion in the environment (which is possible only if there is supra-entropic free energy in the system) and the ability to purposeful behavior (due to surplus information, which is not completely compensated by informational entropy). Based on the idea of an intermediate state of a prebiotic microsystem (protocell) during its transition to life, considered in Section 1.2, the process of the origin of life divides into two periods. The first period is the prebiotic chemical evolution that lasted up to appearance of the intermediate state between non-life and life in the microsystems (at approximately equal balances Sc ≈ FEc, Sic ≈ Ic). The second one corresponds to the primary biotic evolution that proceeded with the onset of TI with a continuous concentrating of supra-entropic free energy and information in living subcells. Its result was the formation of the first living vegetative cells - progenotes, and then prokaryotes.
With a more detailed consideration of the origin-of-life process within the framework of the stated theoretical provisions, the following questions arise: 1) What is a mechanism of the thermodynamic inversion, how does it occur? 2) What adjustments and restrictions does this mechanism introduce into existing ideas about chemical processes in a course of prebiotic evolution and the origin of metabolism? These issues are discussed in the following sections.
Consider the boundary between non-living and living systems, shown in Figure 1, from the point of view of chemistry and thermodynamics. The central part of Figure 1 below shows the main types of prebiotic microsystems—the RNA world, Protein world, Lipids world, and at the top—the root region of the phylogenetic tree of life on Earth, represented by the simplest prokaryotes (Archaea and Bacteria). In the right part of the Figure 1, the main trends are indicated, reflecting the direction of chemical transformations in the course of prebiotic (yellow arrow) and biological (green arrow) evolution. In general, these trends look similar, since in both cases evolution proceeds with the complication of organic molecules, molecular structures and an increase in their chemical diversity. Accordingly, biological evolution looks like a natural continuation of chemical evolution.
A completely different picture is observed on the left side of Figure 1, which shows the opposite thermodynamic trends of chemical (prebiotic) and biological evolution. Here, prebiotic models, like all other non-living chemical systems, exist in a general trend of increasing entropy (brown arrow), which is in accordance with the 2nd law of thermodynamics. At the same time, viable life forms and their communities, including the biosphere as a whole, exist in the opposite trend associated with the concentration of free energy and information (or, what is the same, negative entropy) (red arrow). In this regard, non-living and living systems separate by a thermodynamic, and more accurately by a negentropic barrier (Figure 1, center). From it, they evolve in opposite thermodynamic directions - chemical systems end up on the graph down, with an increase in entropy, and living ones-up, with its relative (in relation to the contributions of free energy and information) decrease. It follows from this that prebiotic systems cannot transformed into primary forms of life simply by gradual chemical complication. This requires their radical thermodynamic transformation associated with overcoming the negentropic barrier. This is the thermodynamic inversion.
The theoretical analysis of the basic scheme shown in Figure 1 leads to the conclusion about the only allowed way of transforming chemical systems into primary life forms, which does not contradict the 2nd law of thermodynamics. First of all, such a transition can only be carried out in an oscillatory mode, through the intermediate state mentioned in the introduction. To start the transition, a chemical system must receive such a powerful impulse of free energy (and information) that far exceeds the current production of entropy in the system (including also its input from outside). Such an impulse (red arrow 1 on the left side of Figure 1) can initiate by abrupt changes in the environment with the corresponding emergence of the conditions far from equilibrium. This impulse must also compensate for the natural trend of the non-living system towards an increase in entropy (brown arrow in the same place), and create a large excess of free energy (and information) in the system, which entropy cannot immediately devalue. For a certain time, such a system exists in a unique and thermodynamically extremely nonequilibrium (unstable) state of entropy deficit, or negentropy excess. The subsequent increase in entropy, inevitable by virtue of the 2nd law of thermodynamics, should return the chemical system to its original state (brown dotted arrow on the left side of Figure 1). But if such powerful impulses are repeated due to periodic strong fluctuations in the parameters in the environment (red arrows 2–3), then such a prebiotic system begins to oscillate around the negentropic barrier, passing into an intermediate state between non-life and life. Being in a position above the negentropic barrier, it has the potential for evolution to life through directed reorganization using the accumulated reserves of supra-entropic free energy and information.
The mechanism of thermodynamic inversion considers in more detail in a number of publications [27,28,29,30]. Briefly, it formulates as follows.
Since a negentropic impulse of great strength can initiated only by sharp changes in the physicochemical parameters in the medium (under equilibrium conditions its appearance is not possible), this refers further consideration of the transient process to the key provisions of irreversible thermodynamics, which considers phenomena under conditions far from equilibrium.
Since a negentropic impulse of great strength can only be initiated by sharp changes in the physicochemical parameters in the medium (under equilibrium conditions, its appearance is impossible), this refers further consideration of the transient process to the key provisions of irreversible thermodynamics, which considers phenomena under conditions far from equilibrium [31,34,35,36,37,38]. If the changes in the environment are strong enough to bring the system out of equilibrium, but not strong enough to destroy it, then it passes from the initial equilibrium state to some new equilibrium state through an unstable bifurcation point. In the region of bifurcations, new properties appear in the system, similar to those observed during some phase transitions. These include phenomena of cooperation (synergism) and heterogeneity of the system due to the presence of sharp energy and concentration gradients, as well as continuous macrofluctuations and exchange of matter, energy, and information with the environment [26,35,37,39]. When the transition of the system to a new equilibrium state is completed, these bifurcation properties disappear.
According to the TI concept, in order to continue evolution to life, a chemical prebiotic system must exist in an oscillatory mode near the bifurcation point, in the so-called "bi-state" state [26,28]. Being in this state, it oscillates between two attractors of comparable strength—the initial and new equilibrium states, neither of which is finally reached. Each transition through this unstable point brings more and more random changes to the system. This is due to the fact that, in accordance with the provisions of non-equilibrium thermodynamics, at the bifurcation point, the tension between the former structure of the system and the emerging new structure reaches a peak, and any slight random change can drastically affect the choice of the direction of its further development. During the reverse transition, some of the changes that have occurred disappear, but the other part preserve, since the system cannot return exactly to the same previous state. Changes at the bifurcation point can lead both to an abrupt increase in the level of organization (order) of the system, and to its radical simplification (degradation). The most important consequence of this for the process of the origin of life is that in the network of chemical reactions in prebiotic microsystems that are in a transitional state between non-life and life, extremely efficient chemical pathways can suddenly emerge. By generating a huge amount of free energy, such transformed networks of chemical reactions strongly shift the balance of "entropy contribution/free energy contribution" in the system to the negative area. As a result, there can be an abrupt evolution (in fact, a revolution) of the system towards life. Conversely, prebiotic systems with spontaneous degradation transformations eliminate from the process of germinal biological evolution and irreversibly fall below the negentropic barrier shown in Figure 1.
It follows from the above that periodic changes in the physicochemical parameters in the medium (including regular and irregular macro- and microoscillations) are not in themselves a sufficient factor for generating a strong negentropy pulse in a prebiotic system. A large flow of free energy into a chemical system is also insufficient if it does not undergo internal reorganization aimed at generating an increasing amount of free energy. In the absence of such a reorganization, this flow will then freely dissipate into the medium with a corresponding increase in entropy in the system. Thus, the key factor is how the prebiotic system reacts to external influences (stress factors), how the total thermodynamic balances "Sc/FEc" and "Sic/Ic" change in it. Being in the intermediate state above the negentropic barrier (Figure 1), the system possess the following possibilities for shifting balances in the negentropic direction, approaching the living state: a) use of supra-entropic free energy and information in the course of internal reorganization; b) appearance of spontaneous structural transformations in the network of chemical reactions arising from oscillations around the bifurcation point. This also implies the great importance of the chemical composition of the initial prebiotic microsystems, which has yet to be determined more precisely. Developing in a negentropic trend, such a system (a cluster of organic microsystems, rather than a separate microsystem) gradually acquires the ability to strengthen its response to periodic external influences (stress factors). This happens due to the increasing prevalence of the contribution of free energy over entropy. It also acquires the ability to purposeful respond due to the increasing prevalence of information over informational entropy (or, one might say, due to an excess of the internal information in relation to the environment). Thus, it begins to show more and more its own activity in relation to the environment and transform it.
Apparently, it is the cluster of microsystems that evolves, similar in the same state near the negentropy barrier, but differing in the amount of free energy. This determines the development of biological properties in each microsystem and their accumulating differences, as well as the acquisition of properties necessary for the survival and evolution of the cluster as a whole. Thus, heterogeneity as a necessary property of a living system arises and evolves simultaneously with the emergence of life and its development.
Possible trends in the further evolution of a prebiotic system, which is in the intermediate oscillatory state between non-life and life, shown in Figure 2. In its upper part (Ⅰ), the evolution of a system is shown in which the ratio "total contribution of entropy (Sc)/total contribution of free energy (FEc)" fluctuates all the time, sometimes spontaneously "jumping" over the negentropic barrier (horizontal axis). During such periods, the system passes into the intermediate state, either rising above the barrier, or falling below it. If it is able to provide an effective (intensified and purposeful) response to the impact of external stress factors, then with each such fluctuation it accumulates more and more supra-entropic free energy, developing along an upward trend towards life (Figure 2, middle part Ⅱ). Such a process is called stepwise activation. Conversely, the inability of the system for effective respond to periodic external influences initiates an irreversible increase in entropy, accompanied by degradation and elimination from the process of germinal biological evolution (Figure 2, lower part Ⅲ). It can be added that, in accordance with the provisions of the theory of stress, external influences can be both beneficial for the system ("stress+") and unfavorable ("stress-") [40].
The importance of changes in the environment for the development of living organisms, including the early stages of the evolution of the biosphere, was also noted by other researchers [41]. The TI concept clarifies this provision in the following way. What is important is not so much the fact of the presence of periodic physical and chemical changes in the environment (the stress impact should not be equal to 0, but should not be excessive), but the nature of the response to them of a cluster of prebiotic microsystems, transforming into a population of primary subcells. The response to the action of a stressor should be enhanced (in relation to the strength of the external impact) and purposeful [26,28].
It follows from the previous section that physicochemical parameters (pressure, temperature, pH, Eh, concentrations of components, electrical potential, etc.) in the environment for the origin of life should fluctuate in various modes with changes in amplitudes, frequencies, periods, etc.). Within the framework of the inversion approach, such fluctuations are considered as the fourth required condition for the emergence of life (in addition to the three generally accepted ones: the presence of organic matter, water, and an energy source). The combination of fluctuations of the listed parameters of different scales is characteristic of hydrothermal systems. But they are not peculiar to the ocean, or the ice sheet, where physicochemical conditions are more or less stable.
In this regard, hydrothermal systems are considered as the most suitable environment for the origin of life, which coincides with the opinion of many experts in the field of astrobiology [12,13,42,43,44,45,46,47]. In recent years, the most active discussion has been between supporters of the origin of life in hot "puddles" on the continents [12,48] and in underwater hot springs at the bottom of the oceans [49]. Note that in both cases, hot solutions come to the surface from deeper regions of hydrothermal systems. Within the framework of the TI model, it is the subsurface environment that is considered as the initial generator of the processes that led to the emergence of populations of primary life forms (subcells). Some scientists have also considered the subsurface regions of hydrothermal systems as the most suitable environment for the emergence of life [43]. In this area, sharp changes in pressure, temperature and other parameters periodically occur (especially during volcanic eruptions and earthquakes). According to the TI approach, just these conditions are necessary for the transition of clusters of organic microsystems (protocells) to a nonequilibrium bifurcation state. Such highly fluctuating conditions in hydrothermal systems are replaced from time to time by periods of relative calm, when low-amplitude fluctuations appear (often referred to as "trembling of the earth's crust"). Such types of oscillations are widely described in the literature on the dynamics of hydrothermal systems [50,51,52,53]. According to the TI model, abrupt changes in the environment contribute to the transition of clusters of organic microsystems to a non-equilibrium bifurcation state (Figure 3). Subsequent periods of low-amplitude oscillations maintain balanced oscillations of microsystems around the bifurcation point, thus providing the possibility of their further evolution to life [26,27]. These conditions also contribute to the continuous reorganization of microsystems and support their interaction in a cluster, as shown in Figure 3.
Another feature of the hydrothermal fluid in subsurface areas is that it migrates irreversibly to the surface against the backdrop of a decrease in temperature and pressure. This is important for the diversification of kinetic traps, the role of which in the course of the emergence of life was considered in detail in the article [54]. Their meaning is that the polycondensation of organic molecules (for example, amino acids) in an aqueous medium inevitably ends with their hydrolysis, that is, decomposition. There is a certain balance between the elongation and hydrolysis rates depending on environmental conditions. It follows from this that the progressive complication of macromolecules, necessary for the process of the emergence of life, will be blocked by increasing hydrolysis. However, as the hydrothermal solution moves up, the conditions in the medium change irreversibly all the time.
When evaluating the subsurface regions of hydrothermal systems and their surface outcrops as potential loci for the origin of life, the following should be emphasized. The conditions in hydrothermal discharges on the continents and on the bottom of the oceans change reversibly: the physicochemical parameters of the hot solution at the point of outflow from the crack fluctuate within limited values with small amplitudes (the latter can increase during volcanic eruptions). In contrast, the parameters of a hydrothermal fluid migrating from a depth of about 1 km to the surface change strognly and irreversibly. For example, in the hydrothermal systems of Kamchatka, the temperature in such a vertical range decreases from values of the order of 200 ℃ to 80–100 ℃, and the pressure decreases from 40–80 bar to 1–2 bar [28,52]. Such a directed change in conditions during the vertical migration of a hot solution, taking into account fluctuations of different ranks of parameters, provides unlimited possibilities for the expansion and irreversible modification of kinetic traps for chemical reactions, when the predominance of the synthesis and polymerization of macromolecules over their destruction. This contributed to the complication and directed evolution of clusters of organic microsystems to life. At the same time, it should be borne in mind that, within certain ranges, an irreversible decrease in the temperature of an aqueous solution in itself contributes to the progressive polymerization of dissolved and colloidal organic matter. Thus, according to the TI model being developed, the hydrothermal system as a whole is the cradle of life on Earth. The already formed or almost formed primary populations of subcells carried out into hot springs (vents), later transformed into the simplest populations of progenotes.
The most probable chemical composition of organic microsystems, which subsequently transformed into the primary forms of life on Earth through the mechanism of thermodynamic inversion, must be determined in the course of prebiotic chemistry experiments in an environment with varying physicochemical parameters (see Section 2.4). The evolution of all relevant prebiotic models proposed by various researchers (or to be proposed in the future) can be experimentally studied under conditions that simulate real fluctuations in hydrothermal systems. But at the same time, some consequences from the thermodynamic provisions of the inversion approach, which are important for prebiotic chemical evolution and early biochemical evolution, must be taken into account.
A membrane with a predominantly lipid composition seems to be a necessary component of the initial prebiotic microsystems. The self-assembly of lipid amphiphilic molecules into a membrane in hydrothermal environments in the context of the origin of life has been discussed in detail in a number of publications [11,12,55]. The membrane formed the discreteness of microloci, where the nascent living system evolved, and fenced off the internal water of the microsystem from the external water of the environment. In addition, the lipid membrane prevented excessive dissipation of free energy in the environment, which was the most important factor for the implementation of thermodynamic inversion.
The lipid membrane should have included molecules of other biologically important components (first of all, polyamino acids, peptides, and proteins), which could ensure the interaction of microsystems both with the environment and with other microsystems in the cluster. Otherwise, microsystems would be isolated and would not be able to start the exchange of matter, energy and information with the environment, as well as in the cluster as a whole. Incorporated macromolecules should provide selective membrane permeability for low molecular weight substances as sources of the main biogenic elements C, N, P, and also support active transport of Na+ and K+ ions against concentration gradients (Na, K-ATPase) during thermodynamic inversion. Such processes provided a pulsating change in the concentration of free water in the microsystem, which affected the rate and direction of reactions.
Another component of the organic microsystem during the TI period was the informational substance (spontaneous sequences of RNA and/or DNA) and polyamino acids (proteins). In particular, this combination of macromolecules is present in the model proposed by Sugawara and Kurihara et al. [9,10]. The continuous interaction and recombination of these different types of (bio)polymers ensured the enormous information capacity of microsystems. This made it possible for them to develop an effective response to external fluctuations, which stimulated their evolution to life [29].
A generalized scheme of an organic microsystem, consisting of the most important molecules for life, considered as the initial prebiotic model, shown on the left side of Figure 4. On the right side of the figure, a prokaryotic cell demonstrates as a model of the simplest living system. On the whole, Figure 4 shows the intermediate position of the same microsystem, which fluctuates between the non-living state (shift to the left) and the primary living state (shift to the right). Obviously, the difference between the left and right parts is not so much in chemistry (the composition of the main chemical components is close), but in the thermodynamic way of their organization. A non-living chemical prebiotic system exists as a passive and subordinate part of the environment fenced off by a membrane, and a tendency towards an increase in entropy inevitably manifests in it. Unlike it, the simplest form of life (a subcell) is isolated from the environment into an autonomous system, it is active in respect to the environment due to the ability to effectively respond to environmental changes, that is, external influences. This thesis correlates with the position of J. Huxley [56], who considered the ability of organisms and communities to actively transform the environment as a key characteristic of life. Accordingly, a living system by its nature is capable of reorganizing its structure, functions and behavior in the environment in such a way as to constantly turn the course of internal chemical and informational processes in the thermodynamically opposite direction (i.e., with the concentration of free energy and information due to a decrease in entropy). The system lives as long as it is able to maintain itself in such a "thermodynamically inverted" state. After TI, it shows a tendency to concentrate free energy and information in primary living systems, associated with the growth of gradients of energy and concentration in them. For biochemical processes, this means that there is a general tendency for synthesis to prevail over destruction, or polymerization over hydrolysis. In modern living systems, this is the first necessary and sufficient condition for the growth and reproduction of cells (organisms), which ensures expansion in the environment.
The temperature ranges for the stability of biologically important molecules (lipids, amino acids, nucleotides, hydrocarbons, and others) in the aquatic environment have been established in a large number of experimental studies and summarized, in particular, in the book [28]. Overall, the findings indicate that hydrocarbons, lipids, and simple amino acids can be synthesized and remain relatively stable in vitro in soluble and insoluble forms over a temperature range of 100–300 ℃, depending on pressure, solution chemistry, and composition of the mineral additives [13,19,20,46,47,57,58,59]. Of course, the synthesis of monomers is in a certain balance with their hydrolysis (what considered above when discussing "kinetic traps"). An emulsion of predominantly hydrocarbon composition may appear as result of their phase separation from water when the fluid temperature decreases to 200–300 ℃ [60]. The temperature range of 150–200 ℃ is suitable for the self-assembly of microsystems of various compositions from lipids and (under very specific conditions) polyamino acids (peptides) if the concentrations of these components reach certain critical values [61,62]. But other important biogenic components—carbohydrates (sugars), nucleic acids, ATP (including their precursors) are stable in a less high-temperature environment. They can be synthesized in vitro at temperatures ranging from 50–60 ℃ (optimal range) to 90 ℃ [7,63,64]. In particular, it is extremely difficult to carry out an abiogenic dehydration reaction in aqueous solutions to obtain condensed phosphates due to the high activity of water. However, in vivo, these components play an important role in the metabolism of prokaryotes, including thermophilic and hyperthermophilic archaea and bacteria that exist at water temperatures from 70–80 ℃ up to 121–122 ℃ [65].
Comparing these data with the most likely environment for the emergence of life on Earth, justified in the previous section (a pulsating updraft of hydrothermal fluid in a vertical interval of 1000 m → 0 m), the following conclusions can be drawn. In the lower part of these ranges, clusters of organic microsystems consisting of lipids, spontaneously synthesized (poly)amino acids, and carbohydrates in their various combinations should have been formed through the process of self-assembly. In this case, the microsystem must have a structured membrane, consisting mainly of lipids. Its composition and structure could become more complex during periods of sudden opening of cracks, accompanied by a rapid drop in pressure and effervescence of the hydrothermal solution. Throughout the ascending hydrothermal flow, oscillatory conditions far from equilibrium should have been maintained in it, which ensured the interaction of organic microsystems in the cluster and contributed to the emergence of primary signs of a living state (the appearance of active transport, an increase of gradients of energy and concentration, etc.). The incorporation of carbohydrates (sugars), ATP, and nucleic acids into this process, accompanied by the formation of nucleoprotein interactions, apparently occurred already in the near-surface regions of hydrothermal systems. In accordance with the developed TI approach, the primary populations of the simplest (sub)microorganisms, formed in this way from clusters of organic microsystems, subsequently began to spread from the outlets of hydrothermal systems in the oceans and/or on the continents (a compromise option is in coastal zones, where both types of thermal outlets are manifested).
To date, almost all experiments in prebiotic chemistry have been carried out under stable conditions. However, several research groups have conducted laboratory experiments and computer simulations under periodically changing conditions: either with temperature fluctuations [10,66,67], or with alternating wet and dry environmental conditions [12]. In the course of experiments, it was found that, in the general case, oscillatory conditions contribute to the polycondensation of macromolecules and accelerate the evolution of prebiotic models compared to stable conditions. This general conclusion was also indirectly confirmed in recent experiments on the synthesis of complex structures consisting of nucleic acid bases as well as amino acids during an ultrafast (1–2 milliseconds) single cycle "shock heating → subsequent cooling" [68,69]. Heating was carried out to the design temperature of 3740–6900 °K at a pressure of 15–34 bar. As a result, various complex structures were spontaneously synthesized, consisting of a mixture of different nucleotides (globule features, twisted filaments and long folded threads).
Considering the results of experiments described above in the context of the inversion concept, we can note the following. The purpose of these experiments was to study the features of the synthesis of biologically important organic compounds, including polymerization and complex formation, under oscillatory environmental conditions. However, from the point of view of the TI concept, more important is to study the response to external fluctuations that develops in prebiotic systems. Experiments of this type have not yet been conducted, and the next section is devoted to a brief summary of the approaches to their implementation.
The general scheme of the proposed laboratory experiments of a new type published in the article [29]. Its essence is as follows. Prebiotic microsystems (models) of different composition and structure are subjected to "pumping" through fluctuations in physicochemical parameters in different modes. It assumes that "pumping" models with external oscillations generates a certain response in them. The "pump" tracks can be different in duration, oscillation mode (amplitudes, periods/ frequencies, etc.), and parameters (pressure, temperature, electric potential, pH, Eh, etc.). The optimal range of periods of regular fluctuations can be from fractions of a second (the rate of induced extremely fast chemical reactions) to 30 minutes (the supposed minimum cell cycle is from division to the next cell division of the most ancient thermophilic prokaryotes). The first step along this experimental path would be to identify the most favorable combinations of prebiotic microsystems and oscillatory tracks that provide signs of an intermediate state between non-life and life. These features include, in particular, the development and increase in the role of active transport, an increase in the degree of homochirality of molecules and the synthesis of macromolecules, etc. During the experiments and upon their completion, the appearance and development of these features could be recorded.
Carrying out the laboratory experiments described in the previous section could test the validity of the theoretical thesis about the induction and stimulation of the origin-of-life process by external influences. However, these experiments would not give an answer to the question of determining the initial biochemical path, which nature actually chose about 4 billion years from the many potential ones. This is a great challenge. Therefore, we propose another way to study the stages of the path that life really went through in the course of its formation.
The following idea put forward as an initial assumption. It known that the ontogenesis of various forms of life repeats phylogenesis. In other words, the succession of events of phylogenesis fixes in ontogenesis, i.e. origin of the properties of the organisms of this kingdom. Both ontogenesis and phylogenesis appeared in the moment of the origin of life. It supposed that the very moment of the origin of life and the initial stages of its development fixed in the initial stages of the ontophylogenetic process of the most primitive forms of life (bacteria and archaea). According to the TI concept, the starting point of life was the intermediate state described above, which combines the properties of both living and non-living systems. In the world of microorganisms, this state most of all corresponds to the state of anabiosis. In the deepest phase of anabiosis, the microorganism, on the one hand, is not alive, since it is unable to counteract actively the increase in entropy. On the other hand, it retains the structural memory of the previous living state, and retains the ability to exit suspended animation with certain changes in the environment.
In order to confirm this assumption, the next Chapter 3 presents the basic data on the state of anabiosis in the world of microorganisms, including the sequence of transformations of a living cell when entering and exiting anabiosis. In the final Chapter 4, the correlation between the given factual data from the field of microbiology on the transition of viable cells to the state of anabiosis and back, and the main provisions of the inversion concept of the origin of life will considered.
The modern concept of anabiosis (synonyms: ametabolism, cryptobiosis, suspended animation) interprets it as a reversible state of the body (part of the body), in which metabolic processes are reversibly sharply slowed down. Experimentally, they are not detected. This contributes to the survival of the organism in adverse environmental conditions (lack of food sources, suboptimal temperature, humidity, etc.). However, initially the concept of anabiosis (Greek-revival) meant the restoration of the vital functions of the body after their absence. This different arrangement of accents demonstrates the presence of two sides of this process: a critical slowdown of metabolic processes up to a complete absence and their restoration with the manifestation of metabolism (exchange of matter, energy and information with the external environment) as a sign of a living system.
The phenomenon of anabiosis as a reversible cessation of the vital activity of an organism was first discovered by Leeuwenhoek about 270 years ago and has since remained one of the most intriguing problems of natural science. This problem was studied most intensively in 60–80 s of the XX century within the microbiological discipline of sporology, where the main model was bacterial endospores. In the field of studying the mechanisms responsible for the acquisition of an anabiotic state by resting forms (RF), there are three groups of hypotheses: (1) the presence (accumulation) of compounds that nonspecifically inhibit enzymes and block cell metabolismn in RF; (2) decrease in the selective permeability of the cell membrane and dehydration (dehydration) of the protoplast; (3) immobilization of cellular biopolymers with loss of their activity [32]. All three mechanisms are united by the fact that they are carried out due to non-covalent weak low-energy physical and chemical interactions. At present, the state of anabiosis as the acquisition by the cell of the metabolic state and exit from it is studied in detail using models of freezing or low-temperature drying of cells in vitro [70,71,72].
The metabolic activity (biochemical reactions) of a cell under favorable environmental conditions for growth is due to the orderly organization and movement of intracellular (macro)molecules and structures, which is ensured by the constant expenditure of free energy consumed by the cell from the environment [73]. Under conditions unfavorable for growth, with the exhaustion of food sources, a criminal change in the physical and chemical parameters of the environment, maintaining the orderliness of the structural organization of the cell in a dynamic way becomes impossible. Then, microorganisms use other physicochemical mechanisms of reorganization of the structural ordering of the cell and changes in the structural organization of intracellular water, in which movement of both intramolecular and intermolecular cytoplasm components (macromolecules and intracellular structures) becomes impossible. The reversible cessation of this movement is the biophysical basis of anabiosis. Such non-volatile mechanisms operate on two levels. At the first level, the formation of temporarily stable intermolecular structures through self-assembly (as in inanimate nature) occurs—this is the biocrystallization of the nucleoid, which occurs due to non-covalent interactions of the low molecular weight Dps protein with DNA [74,75,76]. At the second level—the cessation of their dynamic movement due to changes in the physical properties of the intracellular environment—the cytoplasm. This process, called vitrification, is the glass transition of water in the cytoplasm of the cell to form "colloidal glass". It differs from ice formed by water, whose crystals can damage cell structures [77]. It should be noted that the intracellular space of the cell, the cytoplasm, is an aqueous medium overflowing with low and high molecular weight components [78]. In an aging or dormant cell, the osmotic removal of water from the cytoplasm (under the influence of external factors or during the implementation of cellular mechanisms that appeared in the course of evolution) leads to a decrease in the amount of free water (Aw) to a critical value of less than 70%. As a result, the packing density of macromolecules also increases to a critical level, more than 400 mg/mL, which leads to vitrification of the cytoplasm [77]. Colloidal glass has the properties of a molecular sieve, allowing the passage of small molecules and limiting the diffusion of macromolecules [79]. This property is important for the reversibility of anabiosis. When an anabiotic cell is hydrated (rehydrated) in a fresh medium, small molecules enter it, including those that disrupt the biocrystalline state of the nucleoid, for example, galacturonates [80]. After this brief digression into the biophysics of the cytoplasm of the resting cell, let us turn to the following question.
The state of anabiosis is characteristic of resting (dormant) forms of organisms of different levels of evolutionary development (spores, cyst-like dormant cells; conidia of bacteria and fungi, helminth cysts, plant seeds, etc.). The formation of resting forms is included in the cycle of development of the organism, as an obligatory stage of its ontogenesis (Greek-origin).
According to modern views, a microbial population is considered an organism in the world of microorganisms. Therefore, survival as a property of maintaining the viability of an organism in adverse environmental conditions is inherent only in a population of microorganisms. A microbial population is understood as a set of isogenic (genetically similar) microbial cells localized in space and time interval and forming a complex self-regulating system. The population develops as a single organism and goes through a full cycle of development (ontogeny), which includes several stages [81].
The constitutive property of any population is its phenotypic heterogeneity, that is, the manifestation of functional diversity among genetically identical cells. Thus, only a small part of the population, about 0.01–1.0% of the cells total number, the so-called persister cells [82], have, as the main property (phenotype), the ability to survive in conditions unfavorable for growth. The remaining cells of the population die off (autolyse) when unfavorable conditions occur. The products of their autolysis contribute to the maturation of surviving persister cells into resting, or dormant, forms (RF) that acquire the state of anabiosis [32,83]. Once in conditions favorable for growth, RFs are reactivated, begin to multiply, and again reproduce the parental population, in which a small subpopulation (0.01–1.0% of cells) will again have a survival phenotype. Hence it follows that the necessary conditions for the survival of microorganisms are: (1) a repetitive cyclicity in the development of a population with a change in the stages of growth and dormancy; (2) intrapopulation diversity of phenotypes (properties) of cells, one of which has the properties of transition to a resting state; (3) redundancy in the reproduction of cells as living units. These conditions are necessary for a system acquiring signs of "life".
It should be emphasized that the process of RF formation as a stage in the ontogeny of microbial populations is a chain of events that begins with molecular genetic processes of cytodifferentiation in cells of a small subpopulation (0.01–1.0%). They turn into persister cells with a cardinal change (extreme slowdown) in the type of their exchange of matter, energy and information with the environment. In the chain of these events, the development of anabiosis is only a stage of complete inhibition of the metabolism of the surviving cell as result of physicochemical transformations and structural reorganization of macromolecules and other intracellular components. It ends with vitrification of the cytoplasm. As a result, macromolecules lose their mobility and functional activity, and a cell in a state of ametabolism temporarily suspends metabolic processes and, consequently, vital activity. Thus, in the ontogeny of populations of microorganisms representing the most ancient inhabitants of the planet, the reversible state of their dormant forms anabiosis can be considered as a structural memory of the initial stage of the living state emergence from the inanimate.
The reason for the inclusion of the program for the formation of anabiotic RF is the violation of cell homeostasis, as a stable state of the internal environment of cells, in relation to the perturbing effects of the external environment. The stability of the internal environment of a viable cell is maintained by the balance of synthesis/destruction, while the intensity of synthesis always slightly exceeds the intensity of destruction due to the influx of free energy. This ensures the structural and therefore functional orderliness of the cell to counteract entropy, which is the main functional characteristic of the living.
The disturbing (stress) effect of the environment forces the body to mobilize its physiological capabilities and genetic resources to restore homeostasis, i.e., to form an appropriate adaptive response of cells [32,40,84,85]. This condition is defined as stress. Any environmental factor can have a perturbing, that is, stressful, effect if changes in its quantity go beyond the norm for a given organism and disrupt its homeostasis. If the combination of physiological and genetic capabilities of the population is sufficient to compensate for disturbed homeostasis, the development strategy of the organism does not change, the cells continue to multiply, but at a different rate, the organism adapts to new conditions. This level of change in external factors, that is, stressful influences, is in the zone of tolerance for the body.
With further pressure of stressors beyond the limits of tolerance, the development strategy of the microbial population changes. Cells either die or acquire a new quality that allows them to survive: the genetic survival program activates—the formation of stress-bearing persister cells that mature into resting forms (RF). In this case, the vector of change in stress pressure can be directed towards both its decrease or disappearance (for example, the exhaustion of food sources), and its increase (for example, temperature), as well as the emergence of new stressors (for example, antibiotics). It is necessary to emphasize one more important property of living organisms of any level of organization, including microorganisms, as a prerequisite for their survival. In the absence of stressful effects of the external environment, the system of intercellular communication known as the density regulation system (quarum sensing system, QS-quorum sensing), provides the development of the microbial population staging. One of its functions is the formation of persister cells induction [86].
Spatial self-limitation of population growth, when the number of microbial cells per unit volume reaches a critical value, is perceived by the population as a stressor. Intercellular communication is carried out with the help of low molecular weight substances with the function of autoregulators, which are synthesized by the cells of the population itself and redistributed between the cells. Their threshold concentration in the culture medium is an endogenous signal for the cessation of cell reproduction and space expansion [33,86].
The main categories of stressors as the reasons for changing the development strategy of microorganism populations from their growth to survival are as follows: deficiency of food and energy sources, which violates the positive balance of synthesis/destruction necessary to maintain homeostasis of dividing cells; changes in the physicochemical parameters of the environment beyond the limits of tolerance for a given species; the emergence of new environmental factors as stressors; exhaustion of living space due to the extremely high number of cells per unit volume.
Note, that the imbalance of synthesis/destruction processes, caused by the impact of almost any stressor, is accompanied in the cell by a decrease in the demand for energy equivalents necessary for synthesis processes. This results in imbalance of the electron transfer chain, localized in the membrane, and in accumulation of an excess concentration of reactive oxygen species (ROS) [87]. It stated that almost all stress factors causing disturbances in balanced cell metabolism are accompanied by ROS accumulation. As a result, oxidative stress develops and the systems of enzymatic and, which is important for the problem under discussion, nonenzymatic antioxidant defense of the cell are activated [88]. The result of criminal stress impacts is a change in the development strategy of the microbial population from reproduction to survival, and its transition to the formation of resting forms.
As noted above, the formation of microorganisms RF is a complex multistage genetically determined process in which the acquisition of a dormant state by a cell is the final stage. Preparation for this stage, in addition to new syntheses, includes a number of physicochemical processes that are no longer controlled by the genetic and enzymatic apparatus of the cell. The consequences of these physicochemical processes are: (1) reorganization of biopolymers and cellular structures; (2) inhibition of cell metabolism; (3) acquisition by the cell the property of resistance to stressors.
The general sequence of processes of cell transition to a resting state can be conditionally divided into several successive and irreversible stages.
I stage (initial). It is characterized by cardinal changes at the level of population organization aimed at implementing the strategy of its survival. At the population level, cells differentiate into stress-resistant persisters (~ 1%) and other ordinary cells intended for survival [82]. Under continued unfavorable conditions for growth, ordinary stationary cells autolyze and persister cells mature into resting anabiotic forms, and this autolysate is required for the survival and maturation of persisters [33,83,89]. Thus, the redundancy of cells as living units is necessary for the survival of an organism at any level of organization, starting with a microbial population. This property seems to be so fundamental that one can assume its occurrence even at the moment of the origin of life.
Ⅱ stage. At the cellular and subcellular levels, there are changes in the structural organization of cellular biopolymers—the conformation of enzymatic proteins and the topology of nucleic acids, which leads to an increase in their stability and a decrease in functional activity. For proteins, this effect is achieved due to physicochemical interactions (hydrophobic, electrostatic interactions, intermolecular hydrogen bonds) of protein macromolecules with low molecular weight chemical chaperones (amino acids, phenolic compounds, trehalose disaccharide, etc.), as well as with chaperone proteins [90]. Due to the imbalance of the synthesis processes and the hydrolysis of biopolymers, which begins dominating, concentration of the formed monomers, which function as low molecular weight chaperones, increases. The result of their interaction with protein macromolecules is a decrease in intramolecular interdomain motion in biopolymers [91], which leads to an increase in stability (thermal stability, radioresistance, resistance to Uf-irradiation, etc.) and to a decrease in the functional activity of proteins, up to complete inhibition [33,92,93].
Another cardinal event of this stage is a change in the structural organization of DNA and the nucleoid as a whole, controlled by Nucleoid Associated Proteins (NAPs), the main of which in stationary cells and persisters is Dps (DNA-binding protein from starved cells) [94]. As a result of a number of physical and chemical processes: self-assembly of Dps in dodecamers; their interaction with DNA, the so-called co-crystallization of DNA-Dps; crystallization of Dps-Dps dodecamers - chromosomal DNA is reorganized, the DNA helix iscompressed, and the nucleoid is compacted. A highly ordered structure with crystal-like properties forms—a biocrystalline nucleoid included in the crystal structure formed by dodecamers Dps-Dps-Dps [95]. This reorganization of the nucleoid provides its protection from the action of ROS and hydralases and leads to reversible inhibition of the transcriptional activity of DNA [96]. Such a biocrystalline nucleoid found in resting forms of various types, and was found by us in resting forms from permafrost samples preserved for millions of years [76]. Finally, the protein-synthesizing apparatus of the cell undergoes a structural reorganization: ribosomes (70 S) combine into a non-working structure of doubled (100 S) ribosomes.
Thus, the main processes of this stage are cooperative events leading to the reorganization of the spatial structure of intracellular biopolymers—enzyme proteins, DNA and RNA of the nucleoid, and supramolecular structures—ribosomes, with the loss of their intramolecular mobility and functionality, but with the acquisition of resistance to damaging effects. Let us emphasize the reversibility of these processes, which depends on the water activity (Aw) and the amount of free energy. Similar physicochemical processes, apparently, took place at the prebiotic level of complication and the beginnings of regulation of the organic structures functioning.
During this period, there increases the protection of cellular biopolymers and membrane lipids from oxidation by reactive oxygen species ROS, which accumulate excessively in the cell due to the developing imbalance between energy-giving and biosynthetic processes. Under conditions of a decrease in the activity of antioxidant enzymes (as well as all enzymes) due to their interaction with chaperones, there increases the role of low molecular weight antioxidants, for example, alkylresorcinols, which act as ROS traps and form a system of nonenzymatic antioxidant protection [93].
Ⅲ stage. The structural organization of membranes changes due to an increase in the specific proportion of newly synthesized refractory saturated fatty acids, as well as the incorporation of molecules of chemical chaperones (phenolic compounds, etc.) into the lipid stroma of membranes. The formation of intermolecular hydrogen bonds between the hydroxyl groups of chaperones and the functional groups of lipids leads to a slowdown in the movement of lipids (lateral—along the membrane, and flip-flop—across the membrane). The movement of enzymatic proteins embedded in the lipid stroma also slows down and, thus, the phase state of the membranes changes: their microviscosity increases, their physicochemical properties change; as a result, their functions (barrier, transport and energy-giving) also change, up to their complete inhibition. An imbalance between the synthesis and hydrolysis of membrane lipids, as result of a decrease in the activity of synthases modified by chaperones and inhibition of energy-giving processes, is the motivating cause of autolysis of ordinary stationary cells. At the same time, persister cells, the determining feature of which is resistance to autolysis and lysing solutions, remain intact [97].
Ⅳ stage. The phase state of the membranes changes (microviscosity increases up to polycrystallization), which leads to an increase in their permeability for monovalent ions (K+, Na+) bearing hydrate jackets. Their energy-independent exit from the cell into the environment (gradient diffusion) is the reason for the beginning dehydration of the cell [32]. The onset of dehydration of the cell, in turn, leads to a specific (%) increase in the concentration of the substance in it. A decrease in the amount of free water in the cell creates a borderline situation for the next stage.
Ⅴ stage. The cytoplasm of a resting cell that has matured from a persister is vitrified, i.e., acquires a glassy state (glass former) at a critically low residual amount of free water [77]. This is the final stage in the cascade of physicochemical processes that bring the cell into a state of suspended animation. When the amount of free water decreases to less than 70% and the concentration of the substance is more than 400 mg/ml, there occurs the phase transition "liquid (gel)-liquid (sol)" and formation of a dispersed system, which is a highly concentrated emulsion. This macrophase reorganization of the cell cytoplasm as a polymer-solvent (water) system causes the cessation of the movement of biopolymers and their functioning, i.e., the transition of the cell to the state of anabiosis [72]. Thus, the development of a metabolic block and the acquisition of the state of anabiosis by a resting cell are the result of a gradual physicochemical reorganization of the structure of cellular biopolymers and membranes (as a lipid biopolymer) in their complexes with low molecular weight substances.
As noted above, the metabolic activity of the cell is consistent with the level of its homeostasis as a property of maintaining the internal environment of the cell in accordance with changes in the external environment. Criminal changes in the external environment, that is, stressful influences, disrupt homeostasis and are the motivating reason for the transition of a metabolically active cell to a state of rest or its death. If there is no flow of matter, energy and information in the environment of an anabiotic resting form, then we can talk about the "homeostasis" of an anabiotic cell. In other words, the state of ametabolism of a resting cell is stable in an environment in which there are no changes that disturb the resting state. Such situations exist, for example, in the permafrost soils of the Arctic and Antarctic, the buried soils of the Volga region of Russia, and the burial chambers of the Egyptian pyramids. Under these conditions, when the temporary and reversible inertia of the resting cell corresponds to the inertia of the environment, the state of anabiosis can last for a long time (thousands to millions of years). Cells isolated from these ecotopes remain viable and revert to growth. The duration of the anabiosis state will depend on the increase in the entropy of the anabiotic cell as a set of organic (macro)molecules to a critical level. In other words, a resting anabiotic cell as a "temporarily inanimate system" cannot counteract the increase in entropy.
The reversion of metabolic activity is a fundamental property of RF. The exit of dormant forms from the state of anabiosis and transformation into metabolically active cells occurs in the sequence of events reverse to their transition to the dormant state, includes several stages and has an "explosive" character [32]. Usually there are three stages. The stage of Activation (A) is a reversible period of RF preparation for the beginning of germination (correlates with the final stage V of the cell entering the state of anabiosis). Stage of The stage of Initiation (I) is a period of irreversible transformation of a stress-resistant ametabolic RF into a cell sensitive to environmental factors and metabolically active. At this time, transformations occur that are the reverse of Ⅱ-Ⅲ-Ⅳ stages of the cell entering the state of anabiosis. The stage of Growth (G)—the beginning of the growth cell cycle, leading to the formation of the first vegetative cell, corresponds to the first stage of the cell entering the state of anabiosis. It is important to note that the population of dormant forms is heterogeneous in terms of their ability to revert to metabolic activity in a fresh environment and under appropriate growth conditions: there are fast-germinating RFs, there are slow-growing ones, and there are ones that require additional specific effects for germination.
The motivating cause of the activation stage when the RF leaves the state of anabiosis is the disturbance of rest homeostasis by a free energy impulse, which is perceived as the impact of a stressor, for example, by heating the RF. The main processes of the activation stage are some increase in the degree of fluidity of the cytoplasmic membrane and the manifestation of weak respiratory activity. However, for the release of RF from anabiosis, only the momentum of free energy in the form of heat is not enough. A necessary factor is to maintain this excess energy by supplying sources of energy and nutrition, and information from the environment that these new conditions are suitable for life. Therefore, the activation period is reversible: in the absence of the above environmental conditions, the activated RF returns to the state of anabiosis. Activation considers as the result of physicochemical processes of increasing membrane fluidity and increasing the movement of lipids and membrane proteins. The thermodynamic and kinetic characteristics of these processes are similar to the processes of thermal denaturation of proteins and melting of nucleic acids, but they range from suboptimal to optimal values. If the RF has returned to the state of suspended animation, the RF activation process can repeats, but only a certain limited number of times. The fundamental reversibility and repeatability of the activation of anabiotic RFs, according to the authors, reproduce in microbial ontogenesis the stage of the thermodynamic upheaval of the microsystem with the contribution of free energy to it, according to the hypothesis [28,30].
Analyzing the reversible stage of activation (resuscitation) of an anabiotic cell, we note that the energy-giving process of respiration (as well as heating) that has begun increases the level of free energy in the cell. When the cell returns to the state of anabiosis, this level decreases, but not to its original value, but to a higher one. Therefore, when the activation stage repeats, the anabiotic cell will need less free energy. This phenomenon of "biological hysteresis", similar to the physical one, plays an important role in the germination of dormant forms of modern organisms (spores, cysts and other microorganisms, plant seeds) and is preserved as an atavism in the cycles of their development. Its essence lies in the fact that the return of the excited system to its original state occurs at a level higher than the initial one, that is, part of the introduced energy is preserved. With each new cycle, the level of stored energy increases. If we take into account that the population of any dormant forms is heterogeneous in terms of the degree of dormancy, then the consequence of hysteresis will be an increase in the number of cells returning to viability due to the germination of deeply dormant forms. This technique is used in practice to increase the germination of spore inoculum in microbiological industries and to increase seed germination in crop production. Thus, the process of activation of a resting cell is stepwise, and in this context it can be compared with the process of stepwise activation during the emergence of primary living subcells, theoretically substantiated in Section 2.1.2.
The stage of initiation (I) (divided into three sub-stages—I-1, I-2, I-3), unlike activation, is irreversible. An indispensable condition for initiation is the constant maintenance of excess energy in an animated, dormant form, which ensures by the constant supply of sources of energy, nutrition and information from the environment. During initiation, RF becomes an environmentally sensitive and metabolically active cell.
At the initial I-1 sub-stage, a sharp increase in respiratory activity and devitrification of the cytoplasm is observed due to the generated thermal energy and the beginning of entry into the cell of K+, Na+ ions (along the concentration gradient) that bring free water into the cell. The result of this sub-stage is the cytoplasm watering, which allows the movement of macromolecules and cellular structures in it, and the loss of thermal stability.
At the second I-2 sub-stage, the energy-giving, transport and barrier functions of the cytoplasmic membrane begin to recover. The synthesis of unsaturated fatty acids begins, and the fluidity of the lipid stroma of the membrane increases, which ensures further restoration of its functional activity [98]. In parallel with the restoration of the energy function of the cytoplasmic membrane, but in the absence of synthesis and therefore the lack of demand for energy equivalents (ATP) in the cell, the level of reactive oxygen species increases. The non-enzymatic antioxidant system of the cell neutralize them. The energy generated in the transfer chain e dissipates in the form of heat. Continued watering of the cell and thermal energy are the motivating cause of the destruction of physicochemical interactions between Dps molecules and nucleic acids, as well as between proteins and molecules of both low and high molecular weight chaperones. As a result, the I-2 sub-stage of the PF are flooded, lose their thermal stability and resistance to the action of damaging agents; the permeability barrier is completely restored, as well as the transport and energy-giving functions of the membrane. At the same time, the structure of the protein-synthesizing apparatus of the cell restored, 100S ribosomes decompose into 70 S structures. The synthesis of protein macromolecules is still lacking [32].
At the sub-stage I-3, degradation processes of hydrolysis of proteins and IF-specific structures develop under the action of hydralases released from chaperones and restoring their structure. The resulting amino acids are used for the synthesis of some enzymatic proteins, for which transcription templates of messenger RNA, the so-called "stored transcripts" [99], have been preserved in RF.
Thus, as result of the initiation stage, a resting cell irreversibly transforms into a vegetative cell with a completely restored subcellular structure, which is sensitive to environmental factors and metabolically active, with powerfully pronounced degradation processes and partial release of hydrolysis products from the cell. There is no complete synthetic activity due to the unpreparedness of the information apparatus of the cell [32].
The next stage of Growth is the final period following initiation. It is part of the growth (cell) cycle leading to the formation of the first mature vegetative cell. First, the genetic apparatus of the cell as its information basis is completely restored: replication of nucleotide DNA begins with parallel syntheses of informational and transfer RNA, as well as the synthesis of new ribosomes. Following this, the sequential syntheses of enzymatic proteins ensure active cell metabolism, and the predominance of synthesis processes over hydrolysis processes leads to cell growth and an increase in its volume. Upon reaching a critical size, the cell divides into maternal and daughter.
The fundamental material presented in Sections 3.1–3.6, obtained in experimental studies of dormant forms of microorganisms, allows us to formulate a number of the following key provisions that are important for explaining the requirements for the conditions for the emergence of life. Obviously, they must be taken into account by any concept that offers its own explanation for the appearance of life on Earth.
A. In the development and existence of any biological system at all levels of its organization, including a population of microorganisms, two opposite tendencies are manifested: to cooperation, or integration (for example, cooperation of cells into a population as a single surviving organism); to heterogeneity (for example, the differentiation of a microorganism population into separate subpopulations of cells—phenotypes—with differing properties).
Heterogeneity manifests itself at several levels. At the level of phenotypic differences (i.e., differences in cell properties), the population differentiates into a small subpopulation of persister cells that are resistant to autolysis and mature in RF, with concomitant autolysis of the main part of the population, ordinary cells. At the level of RF heterogeneity in terms of the depth of their dormancy, differences are revealed in the degree of their stress resistance (thermal resistance) and the ability to quickly germinate or, conversely, the requirement for this special conditions. At the subpopulation level, the same trends can be traced. For example, in a subpopulation of dormant forms, their heterogeneity in terms of thermal stability and depth of dormancy manifests. A very important manifestation of the heterogeneity of RFs matured from persister cells is their ability to germinate as a mixed population of colonies of various phenotypes, which differ, among other things, in physiological characteristics. All this means that already during the period of transformation of the prebiotic system (cluster of protocells) into the primary living system, a quantitative and qualitative hierarchy of properties (features) of its members was formed.
B. The stability of the organization of a viable (microbial) cell is maintained by the balance of synthesis/destruction of organic matter when the intensity of synthesis always somewhat prevails. Since in the general case the contribution of free energy is necessary for synthesis, and destruction is associated with an increase in entropy, the existence of a prebiotic system and its development into primary life forms (subcells, it follows that progenots and prokaryotes) was ensured by a positive balance "total contribution of free energy > total contribution of entropy" at the early stages of biological evolution on Earth.
C. A high content of free water (Aw > 70%) in the emerging living subcell is a prerequisite for the intermolecular and intramolecular movement of macromolecules that catalyze enzymatic reactions at high rates. An example is the rehydration (watering) of a dormant form, which is a resolving factor for the active action of hydrolases at the stage of RF initiation. On the other hand, a decrease in the amount of free water (Aw), and, accordingly, an increase in the protocell density, induces physicochemical interactions of macromolecules with small molecules or other macromolecules. This leads to a decrease and then cessation of intramolecular interdomain motion and functional activity of macromolecules. For example, reversible interactions of enzyme proteins with low molecular weight chaperones or chaperone proteins lead to inhibition of the catalytic activity of enzymes.
D. In the evolutionary complication of organic matter, the formation of biopolymers is the most important stage in the process of the origin of life. In this context, physicochemical interactions of biopolymers with low molecular weight compounds is defined as a mechanism for regulating intramolecular interdomain movement and, consequently, their functional activity. In this case, the structure and concentration of low molecular weight substances will determine the vector and level of changes in intramolecular motion in biopolymers. Accordingly, the quality of their response (stimulation or inhibition) to extreme environmental changes will depend on this. It assumed that this primitive, primary level of organization of the "stress response" of biopolymers began to emerge in the course of prebiotic evolution. In modern microorganisms and organisms of a higher level of evolutionary development, it preserved as an atavism.
The presence of stressors, i.e., disturbing influences of the external environment, is a necessary condition both for the induction (beginning) of the transition of a microbial population to survival in the form of dormant anabiotic forms, and for their exit from the state of anabiosis. The transition itself carried out through the active response of the microbial population to stress. The stressor for these transitions can be energy intake into the system and its maintenance, or, conversely, the loss of energy in the system. Anabiosis can be seen as an intermediate state of a dormant form between life and non-life.
In the next chapter, it considered the validity of the analogy between this local starting point of the anabiotic form reversion to life activity and the universal starting point of life emergence on Earth, that is, the moment of the emergence of simplest life forms (living subcells).
The TI concept presented in the section 2 considers in general terms: a) the thermodynamic direction of the process of the origin of life (Section 2.1); b) conditions in the parent environment (Section 2.2); c) some aspects of the initial chemical composition of prebiotic microsystems (Section 2.3); d) an intermediate state between clusters of prebiotic microsystems and populations of living subcells, . In general, the process of the emergence of life, according to the TI approach, proceeded in the following succession: prebiotic microsystems → protocells → living subcells (probionts) → simplest vegetative cells (progenotes) → prokaryotes. Within this broad framework, a wide range of specific chemical pathways potentially possible at the origin of life can be considered and substantiated in more detail. But is it possible to determine which of the many possible sequences of chemical transformations actually took place during the emergence of life on the early Earth? The article substantiates that such a sequence of chemical-biochemical pathways at the beginning of metabolism was fixed at the earliest stages of the ontophylogenetic process, and now manifests itself in the process of the exit of a bacterial cell from anabiosis. Starting from the period of the origin of metabolism in prebiotic microsystems, this succession is repeated with some variations in the existence of microorganism populations. The stages of the succession of processes when a cell enters and exits from anabiosis are confirmed experimentally in the theory of anabiosis. We build them into the TI concept of the origin of life on Earth. The basis for such a correlation is the compatibility of the TI concept and the theory of anabiosis in terms of key provisions, which considered in the previous chapters of the article and briefly summarized in the next section.
The correlation between the theory of anabiosis (suspended animation) and the TI concept is based on four generalized regularities that manifest themselves in the foundation of life: the existence of an intermediate state between non-life and life as the initial state for the transition to life; stepwise activation as an energy process for the system to acquire a living state; the prevalence of synthesis over hydrolysis during this process; manifestation of contradictory tendencies towards heterogeneity and cooperation. All of them are substantiated both in the theory of anabiosis and within the framework of the TI concept. These regularities briefly discuss below.
One of the fundamental provisions of the TI concept in the context of comparison with the theory of anabiosis is the following: in order to start life, a prebiotic system must receive a powerful impulse of free energy (as well as information) initiated by a sharp change in the environment. The repetition of such impulses brings the system into an oscillatory intermediate state between non-life and life (Section 2.1; Figure 1, left side; Figure 4, center). This state is reversible: from it the system can both start the transition to life through stepwise activation (Figure 2, Ⅱ; Figure 4, right side), or return back to the prebiotic state with an irreversible increase in entropy (Figure 2, Ⅲ; Figure 4, left side). The anabiotic state of a bacterial cell also interprets by us as intermediate between living and non-living (Section 3.2). On the one hand, an anabiotic cell cannot counteract the increase in entropy due to the suspension of metabolic processes; on the other hand, it retains a structural memory of previously active biochemical processes, which allows it to emerge from anabiosis upon the onset of favorable external conditions. The beginning of the exit is associated with the receipt of an impulse of free energy, which perceives as the impact of a stressor (Section 3.6).
According to the TI concept, the transition of a prebiotic system from an intermediate to a living state occurred against the background of a tendency to concentrate surplus (supra-entropy) free energy and information associated with an increase in energy and concentrations gradients in it (Section 2.1; Figure 2, Ⅱ). For biochemical processes, this means that there is a general tendency for synthesis to prevail over destruction, or polymerization over hydrolysis (Section 2.3). The presence of the same tendency in the process of reviving an anabiotic resting form and functioning of a living cell, in which the intensity of synthesis always somewhat exceeds the intensity of destruction due to the influx of free energy, confirmed by numerous data in the field of microbiology (Section 3.3).
Another fundamental tenet of the TI concept is the presence of conditions far from equilibrium in the environment for the origin of life, which ensure the flow of this process in a nonlinear bifurcation region (Section 2.1). Contradictory tendencies towards cooperation (synergism) and heterogeneity (sharp energy and concentrations gradients) are typical of such highly nonequilibrium chemical systems. The presence of the same tendencies at different levels of organization—from molecular to population—in already formed living systems (Sections 3.2, 3.4) emphasizes the fundamental compatibility of the TI concept and the theory of anabiosis.
The above formulated is the basis for considering the stages of the formation of metabolism in the following sections.
Based on the comparison of the inversion approach with the theory of anabiosis, four stages of metabolism emergence in a cluster of prebiotic microsystems migrating with a hydrothermal fluid to the surface against the background of a pulsating decrease in temperature and pressure were identified: 1) the stage of self-assembly of a cluster of prebiotic microsystems from dispersed organic matter in a hydrothermal fluid; 2) the stage of activation: the transition of microsystems to an oscillatory intermediate state between non-life and life, the formation of protocells; 3) the stage of initiation: the appearance of the simplest living subcells (probionts); 4) the stage of growth: formation of primary living cells (progenots). Since the stages of cell exit from anabiosis—activation, initiation and growth—serve as a structural basis for further consideration of the development of metabolism, these names are retained in the stages of the modified TI concept.
It is known that the self-assembly of organic matter dissolved in water into microsystems of various compositions largely depends on its concentration. Due to the homogeneity of the ancient ocean, self-assembly of microsystems in it would require a huge amount of dispersed organic matter, which may have been absent during the period of the origin of life on Earth. However, hydrothermal systems are heterogeneous, and the appearance of high concentrations of organics in its local areas is quite realistic. The principal mechanism of concentration of organic compounds in periodically opening and closing fracture systems (due to intense volcanic and tectonic processes) during fluid migration to the surface is considered in the book [28]. Its essence boils down to the fact that when a crack closes in a certain area of the hydrothermal system, a lighter organic fraction begins to accumulate under the formed "plug", and when a critical concentration is reached, it forms a cluster of organic microsystems. After the opening of this crack, the cluster with the solution migrates upward and begins to evolve. In this case, due to the rapid drop in pressure, as well as a slower decrease in temperature, strong fluctuations of physicochemical parameters occur, up to the separation of the fluid into liquid and vapor-gas phases. Such changes maintain conditions far from equilibrium in the environment, and contribute to the rapid evolution of clusters along diversified trends. In addition, at certain values of parameter fluctuations in the medium, self-assembly can proceed in an oscillatory (reciprocating) mode, which, under conditions far from equilibrium, was another factor in the diversification of the composition of microsystems and the chemical reactions occurring in them.
The chemical composition of organic microsystems at the stage of spontaneous self-assembly of the cluster should be in accordance with the physicochemical parameters of the hydrothermal fluid at this initial stage: depth starting from 1 km and less, temperature below 200 ℃, but much higher than 100 ℃ (100 ℃ ≪ T < 200 ℃). The available theoretical and experimental material indicates that prebiotic microsystems self-assembled under such conditions must have had a lipid membrane (Section 2.3). Liposomes as initial prebiotic models in a high temperature environment have been proposed in a number of works [11,12,54]. Based on the data from the theory of anabiosis, it should be added that the initial period of the revival of a resting cell is associated with changes in the cytoplasmic membrane (some increase in its fluidity). This fact emphasizes the presence of membrane structures at the base of the process of the emergence of life.
Natural hydrothermal solutions are multicomponent systems in which many other organic compounds will inevitably participate in the process of self-assembly of liposomes. For example, a sterile condensate of a steam-water mixture from a deep well in Kamchatka (depth 2 km, temperature 170 ℃, pressure 8.1 bar, pH 4) contains 48 organic compounds of medium volatility belonging to 9 homologous series [28,53]. Most of them are aromatic hydrocarbons (with one or more benzene rings), normal alkanes and their isomers. Isoprenes, sulfur-containing and oxidized hydrocarbons (aldehydes, ketones, alcohols) are also defined here. A large role of aromatic hydrocarbons in the early stages of life emphasize by a number of researchers [7]. According to our data, in general, there is a trend towards an increase in the diversity of organic compounds with a decrease in the temperature of the hydrothermal fluid [28,53]. However, a significant number of simple organic compounds (alkanes, aromatic hydrocarbons and their sulfur-containing homologues) also found in hydrothermal fluid with a temperature of 300–350 ℃ [100]. All this points to the complex composition and discrete structure of the initial prebiotic microsystems, which were based on liposomes. Among other biologically important compounds, (poly)amino acids were probably present in the original microsystems. They can exist at high temperatures up to 200 ℃ and even higher. Their presence in natural fluid with temperatures up to 120 ℃ is confirmed [13,20,28,43,101]. Although amino acid oligomers show a tendency to degrade in an aqueous medium, their synthesis can be maintained by a minimum amount of free water inside liposomes and diversification of kinetic traps during pulsatile fluid migration to the surface. Other biologically important components—(poly)nucleotides, ATP and sugars were most likely absent at this initial stage of the prebiotic process due to the high temperature in the medium (100 ℃ ≪ T < 200 ℃).
According to the theory of anabiosis, the stage of activation of a resting cell consists in the appearance in it of a weak energy-giving process of respiration, which (like the influx of heat from outside) increases the level of free energy in it (Section 3.6). In the context of the above analogy, the same beginning is provided for the stage of activation of a cluster of prebiotic microsystems migrating in a pulsating hydrothermal flow to the surface. One way to supply additional energy to microsystems is the periodic supply of heat associated with temperature fluctuations. Also, under the conditions of the existence of various physicochemical gradients (both vertical—from the depth to the surface, and horizontal—from the axial zone of the flow to the periphery of cracks), electrons transfer inevitably occurred in the cluster, which supported redox reactions in the microsystems. The presence of such gradients considers in [28,102]. The most important role of electron transfer under conditions of sharp geochemical gradients for the origin of life also substantiates in the submarine alkaline vent theory of the origin of life [15,49,103]. According to this approach, the origin of life occurred due to the deposition of organic compounds in mineral chimney precipitations under nonequilibrium conditions between the ocean and vent solutions, which is very different from the course and place of the process proposed by us. But what is common is that the primary activation of prebiotic microsystems occurred due to redox reactions associated with the transfer of electrons from a donor to an acceptor. The same conclusion was made by Hengeveld and Fedonkin in the framework of their own reconstruction of energy transfer in the metabolic network: 1) redox reactions associated with the transfer of electrons from a donor to an acceptor were the original ones; 2) acid-base reactions of phosphates associated with proton transfer appeared later [104,105].
Over time, in the course of an increase in the level of free energy in the cluster and in individual organic microsystems that had a heterogeneous structure due to far from equilibrium conditions in the environment, loci arose in which there was an excess accumulation and generation of free energy in the course of chemical reactions. Such loci referred to in the scientific literature as "low-entropy structures" [28,106]. In such loci, excess free energy appeared, which not fully compensated by entropy due to rapidly occurring nonequilibrium processes in the medium initiated by fluctuations. With strong, but optimal external changes, due to the presence of excess (supra-entropy) free energy in such loci, an enhanced response of the system to external influences was born, overcoming the pressure of entropy. The article considers such a response as a "negentropic" impulse, due to which the prebiotic system could overcome the negentropic barrier (Chapter 2.1; Figure 1, left side). Such a negentropic impulse was transmitted from one cluster's microsystem to another, laying the foundation for the emergence of biogeochemical interactions and biogeochemical cycles (Figure 3, red arrows). Some microsystems generated and transferred to others in the cluster a large amount of excess free energy (as well as information), maintaining the integrity of the emerging primary population.
According to the theory of anabiosis, the reversible stage of activation begins with local watering of the shells of dormant forms, accompanied by some acceleration of the movement of lipids and membrane proteins. This gives grounds to believe that at the stage of activation, at the inception of metabolism, there was also a certain acceleration of the movement of lipids in membrane structures, associated with an increase in their fluidity. In the same context, one can also assume an acceleration of the rate of (bio)chemical reactions occurred in the membrane. At the same time, it should be emphasized that the key moment in the emergence of metabolism (and life as a whole), according to the inversion concept, is not just the flows of various chemical processes (as they occur in various chemical systems), but the active response of the system as a certain integrity to external influences. The nascent living system began to organize and build for the perspective (using the ability to purposefully respond) all chemical processes in such a way as to ensure its existence and development at the negentropic level located above the thermodynamic barrier (as shown in Figure 1 and Figure 2, Ⅱ). At the same time, the accumulation of information at this stage occurred mainly due to the fixation of traces of external influences in the composition, structure, and configurations of molecules in the system [28,107,108]. In fact, it was complex information about the outside world that entered the microsystem and imprinted in it. Its own information about the processes occurring inside the nascent subcell and characterized by a trend towards a decrease in the share of informational entropy began to accumulate already in the next stage with the development of the process of thermodynamic inversion in it. Such supra-entropy information, associated with the active response of the system to external influences, already had the property of purposefulness, in accordance with which the subsequent reorganization of the system took place. It considers in the article as the initial proper biological information. With the evolution of the purposefulness property, the emergence of prescriptive information (instructions) is also associated, including any form of programming. The most important role of prescriptive information in the process of the emergence of life especially emphasized by D. Abel [109].
The transition from the activation stage to the next stage of initiation began when the stepwise activation of the cluster of prebiotic microsystems (protocells) reached a critical level, beyond which this process became irreversible (although still very unstable). This means that such a system was able to maintain such a level of free energy generation and information accumulation that provided it with control over entropy. Within the framework of the concept of thermodynamic inversion, such a relationship between free energy, information and entropy considers as the main criterion for distinguishing biological (living) systems from non-living chemical systems. Since the prebiotic system (protocell) at the activation stage thermodynamically corresponded to the transition state between non-life and life (Sc ≈ FEc, Sic ≈ Ic: see Section 2.1), its subsequent development occurred against the background of a shift of these balances to the negentropic region (Sc < FEc, Sic < Ic). Such thermodynamic changes in the system corresponded to the reorganization of (bio)chemical processes and the formation of metabolism already in the next stage of initiation. That is, in fact, the stage of initiation in the formation of metabolism coincided with the period of thermodynamic inversion, during which the contributions of free energy and information in the system changed from comparable to the contribution of entropy (initial "zero point") up to dominant. Thus, in the course of initiation, a system arose that can be defined as the simplest population of living subcells. In a number of previous publications, such subcells designated as "probionts" [28,107]. Living cells that possessed the primary genetic apparatus were formed later at the final stage of growth.
Following the stated above correspondence between the inversion concept and the theory of anabiosis, the stage of initiation in the process of forming the metabolism of a living cell divided into three sub-stages—early (I-1), middle (I-2) and late (I-3). In general terms, this sequence coincides with the subdivision of the initiation stage when a dormant bacterial cell emerges from anabiosis into three sub-stages (I-1, I-2, I-3, respectively), which was considered in Chapter 3.6.
According to the theory of anabiosis, at the initial sub-stage (I-1) there was a sharp increase in respiratory activity and devitrification of the cytoplasm due to the generated thermal energy and the beginning of the entry into the cell (along the gradient of concentrations) of K+, Na+ ions, introducing free water into the cell. Similar changes occurred in nascent subcells, according to the approach developed in the article. Energetically, this process provided by the rapid accumulation of supra-entropy free energy in the nascent populations of subcells, supported by the ongoing stepwise activation. The result of this early sub-stage was the watering of the (proto)cytoplasm, accelerating the movement of macromolecules and subcellular structures in it. Polynucleotides at this stage should probably have been absent in the environment due to the high temperature of the solution (which, according to geological data, should have exceeded 100 ℃), but their local synthesis within subcells is not excluded.
In the middle sub-stage (I-2), watering of the subcells continued. At the same time, the thermal stability of subcells and their resistance to the action of damaging agents decreased. The synthesis of unsaturated fatty acids began. The permeability barrier began to form, as well as the transport and energy-giving functions of the membrane. Simultaneously with the increase in the energy function of the membrane, the level of reactive oxygen species increased, for the neutralization of which a non-enzymatic antioxidant system of the subcell formed. At the same time, the foundation of the primary structure of the protein-synthesizing apparatus of the subcell took place, but the matrix synthesis of protein macromolecules was still absent. Energy equivalents (ATP) in the absence of biosynthesis have not yet been in demand.
In the late sub-stage of initiation (I-3), the accumulation and reorganization of bioinformation in subcells reached a critical level. Bioinformation in the article in a broad sense means information that is characterized by the balance Sic < Ic, which ensures the ability of life forms to purposefully and taking into account the perspective to respond to external influences. During this period, an intensive process of formation of informational macromolecules began, which stored information about itself in the subcell, and thus supporting its existence as a unique unit of life. Following the theory of anabiosis, during this period one can assume the appearance of primary RNA templates that took part in the synthesis of a number of enzymatic proteins. It is assumed that at this sub-stage the temperature in the medium was even higher than 100 ℃; therefore, nucleotides and their sequences should have appeared in subcells mainly as result of internal targeted synthesis, and not due to input from outside. This process proceeded simultaneously with the release of amino acids due to protein hydrolysis under the action of hydrolases. Around the same time, acid-base reactions of phosphates associated with the transfer of protons began to dominate in energy conversion processes and led to the widespread use of ADP and ATP. The diversification of kinetic traps associated with an irreversible drop in temperature and pressure during the migration of hydrothermal fluid to the surface facilitated biosynthesis in subcells, preventing hydrolysis.
The stage of initiation in the process of the origin of metabolism considers in the article as irreversible, as well as the stage corresponding to it in the theory of anabiosis. Deterioration of conditions for the existence of populations in the environment naturally triggered either the reverse process of the return of subcells to the initial intermediate state between non-life and life (ie, to anabiosis), or their destruction. In the first case, due to the appearance of interacting informational molecules, the subcells could no longer be completely destroyed during the transition to a passive state (suspended animation). As follows from the theory of anabiosis, "stored transcripts" of RNA should have been preserved, which could be used for protein synthesis when external conditions change and the cells of the population emerge from anabiosis.
As mentioned earlier, the last stage of growth in the process of the emergence of life is the reverse of the early (1) stage of the transition of a modern cell to a resting form. During this stage, the formation of the growth cell cycle took place, which led to the appearance of the first living vegetative cells, as constituent elements of the primary populations. At this time, the genetic apparatus of the cell began to form as its informational basis. The targeted synthesis of enzymatic proteins that followed ensured active cell metabolism. The significant prevalence of synthesis over hydrolysis led to cell growth and an increase in their volume. Upon reaching a critical size, the cell organically divided into maternal and daughter cells, which was facilitated by the initial thermodynamic bifurcation of the prebiotic system under conditions far from equilibrium (the so-called "bi-state" state, see Section 2.1.2).
The primary cells formed during the growth stage correlate in the article with "progenotes". The progenote as the earliest and already extinct form of life theoretically reconstructed by C. Woese [24]. Organisms of this type could have had a genotype and phenotype (i.e., information stored in a quiescent [replicative] form in one class of molecules that was also manifested in an active [functional] form in another), but their genes would for the most part have been physically separate units; they would not be organized into large contiguous linear arrays. Proteins would have been small or nonunique sequence or both. It follows of the reason that proteins of normal size could not have been synthesized without introducing (many) errors. The consequence is that their enzymes could not be as accurate and specific as their modern counterparts. The progenote reasonably had error rates two or three orders of magnitude greater than found in cells today. To keep existence of minimally functional cells, genes should be disjoint, and they could have existed in high copy numbers.
The completion of the formation of the primary progenotes' populations occurred when the hydrothermal fluid flows reached the near-surface regions. The most optimal is the vertical range from the outlets of hot springs on the continents and/or the bottom of the oceans to depths of several tens of meters. The scale of physicochemical fluctuations (amplitudes, frequencies/periods) of hot solutions and steam-water mixtures in these zones noticeably decreases, which confirms by monitoring data in various geothermal regions. The temperature regime was in the range of 100 ± 20 ℃. The lower limit of 80 ℃ is determined based on the features of the process of polycondensation of macromolecules, data on microbial ecology, as well as the ocean temperature calculated from isotope data in the range of 3.6–3.8 billion years ago (values of 70–80 ℃ prevail) [110]. The upper limit of 120 ℃ corresponds to the maximum growth temperature of some modern types of hyperthermophilic microorganisms (121–122 ℃) [65]. After the release of the primary populations of progenotes into the ocean, their long evolution began, which led to the appearance of prokaryotic cells of archaea and bacteria. According to the reconstruction by Woese [24], evolution of progenotes up to prokaryotic cells proceeded through significant rise of a level of the translation accuracy, elongation of strings of the both classes of biopolymers, the formation of DNA and a genome.
The authors are very grateful to Alla Voronina and Elena Erofeeva for assistance during preparation of the manuscript for publication.
V.N.K: sections 1.1, 1.2, 1.4, 2, 3.7, 4; G.I.E-R: sections 1.3, 1.4, 3.1–3.7.
The authors declare no conflict of interest.
Anabiosis (suspended animation): the reversible cessation of vital activity of an organism;
Autolysis: self-dissolution of the cell under the action of its own enzymes—depolymerases of cellular polymers;
Chaperones: 1) molecular—proteins that stabilize the structure of enzymatic proteins; 2) chemical—low molecular weight compounds (amino acids, carbohydrates, etc.), stabilizing and modifying the structure (tertiary, quaternary) of enzymatic proteins, which affects (inhibits or activates) their functional activity;
Entropy (S): both a measure of lowly valuable energy (it is opposite of free energy) and a measure of system disorganization (it is opposite of information); the part of entropy responsible for informational processes designates as Si;
Free energy (FE): a measure of highly valuable energy (the part of the inner energy of the system that can be converted into work of any kind);
Information (I): a quantity that reduces the uncertainty of the state of a system;
Kinetic trap: stable prevalence of synthesis (polymerization) of organic molecules over hydrolysis in increasingly complex prebiotic (micro)systems, which is achieved by changing the physicochemical parameters of the aquatic environment in the optimal mode;
Negentropy is a negative entropy, which in its meaning consists of the contributions of free energy and information to a natural system;
Negentropic barrier: a thermodynamic boundary separating non-living and living systems, that defines the impossibility for non-living systems to continuously maintain the presence of supra-entropic free energy and information (due to their active extraction from the environment and internal reorganization);
Prebiotic microsystem: an organic microsystem considered as potentially suitable for the transition to life, but which lacks two key properties of the living state—the ability to expansion in the environment and purposeful behavior (stage 1 of the origin of life in the IT concept);
Protocell: a prebiotic microsystem that is in an oscillatory intermediate state between non-life and life (stage 2 of the origin of life in the IT concept);
Probiont: the simplest living subcell with the presence of interacting informational molecules (RNA and proteins), in which two key properties of the living state are manifested—the ability to expansion in the environment and purposeful behavior (stage 3 of the origin of life in the IT concept);
Progenote: the earliest extinct life form with a mature genetic apparatus theoretically reconstructed by C. Woese; within the framework of the TI concept, it correlates with primary living vegetative cell (stage 4 of the origin of life);
Resting (dormant) forms of microorganisms (RF): cells designed for long-term (months-millions of years) survival in non-growth conditions: ametabolic, stress-resistant, characterized by ultrastructural organization.
Reactive oxygen species (ROS): formed in reactions of one-electron reduction of oxygen, therefore they are able to oxidize biological molecules;
Persisters: a small (0.01–1%) subpopulation of vegetative bacterial cells, extremely slowly dividing, surviving lethal doses of antibiotics, possible precursors of dormant forms;
Supra-entropic (supra-entropy, super-entropy) free energy: a surplus part of free energy that remains in living systems after full compensation of entropy; this ensures their ability for expansion in the environment;
Supra-entropic information: a surplus part of information that remains in living systems after full compensation of informational entropy; this ensures their ability for purposeful reorganization and behavior in the environment;
Thermodynamic balance: it determines the overall thermodynamic direction of chemical and other processes in a natural system at the macroscopic level. There are two key balances: "Sc/FEc", where Sc is the total contribution of entropy, and FEc is the total contribution of free energy into the system; "Sic/Ic", where Sic is the total contribution of informational entropy, and Ic is the total contribution of information into the system;
Thermodynamic inversion: a radical transformation of a prebiotic (micro)system, during which chemical processes in it begin to proceed in a thermodynamically opposite direction, which determines its transition to a living state;
TI (thermodynamic inversion) concept: the original approach by V. Kompanichenko, according to which the transition to life carries out through thermodynamic inversion due to intensified and purposeful response of prebiotic microsystems to physic-chemical oscillations in the environment (stress).
Stepwise activation: a tendency to increase the level of free energy in a prebiotic microsystem or a bacterial cell emerging from suspended animation in a reciprocating mode;
Vitrification (glass transition) of the cytoplasm: its transition from a gel state to a sol due to a critical decrease in free water; characteristic of resting cells.
[1] | Oparin AI (1957) Origin of Life on the Earth. Moscow: Nauka. |
[2] | Fox SW, Bahn PR, Pappelis A, et al. (1996) Experimental retracement of terrestrial origin of an excitable cell: Was it predictable? Chemical Evolution: Physics of the Origin and Evolution of Life, Basingstoke, UK: Springer Nature, 21-32. https://doi.org/10.1007/978-94-009-1712-5_2 |
[3] |
Ikehara K (2015)[GADV]-Protein World Hypothesis on the Origin of Life. Orig Life Evol Biosph 44: 299-302. https://doi.org/10.1007/s11084-014-9383-4 doi: 10.1007/s11084-014-9383-4
![]() |
[4] | Joice GF, Orgel LE (1993) Prospects for understanding the origin of the RNA world. The RNA World, New York: Gold Spring Harbor laboratory Press, 1-25. |
[5] | Deamer DW (2004) Prebiotic amphiphilic compounds, In: Seckbach J, Origins. Cellular Origin, Life in Extreme Habitats and Astrobiology, Dordrecht, The Netherlands: Springer, 75-89. https://doi.org/10.1007/1-4020-2522-X_6 |
[6] |
Luisi PL (2000) The relevance of supramolecular chemistry for the origin of life. Adv Supramol Chem 6: 287-307. https://doi.org/10.1016/S1068-7459(00)80009-0 doi: 10.1016/S1068-7459(00)80009-0
![]() |
[7] |
Ehrenfreund P, Rasmussen S, Cleaves J, et al. (2006) Experimentally tracing the key steps in the origin of life: The aromatic world. Astrobiology 6: 490-520. https://doi.org/10.1089/ast.2006.6.490 doi: 10.1089/ast.2006.6.490
![]() |
[8] |
Huber C, Wächtershäuser G (1998) Peptides by activation of amino acids with CO on (Ni, Fe)S surfaces: implications for the origin of life. Science 281: 670-672. https://doi.org/10.1126/science.281.5377.670 doi: 10.1126/science.281.5377.670
![]() |
[9] |
Kurihara K, Tamura M, Shohda Ki, et al. (2011) Self-reproduction of supramolecular giant vesicles combined with the amplification of encapsulated DNA. Nature Chem 3: 775-781. https://doi.org/10.1038/nchem.1127 doi: 10.1038/nchem.1127
![]() |
[10] | Sugawara T, Kurihara K, Suzuki K (2012) Constructive approach toward protocells. In: Mikhailov A, Engineering of chemical complexity, 1-17. https://doi.org/10.1142/9789814390460_0018 |
[11] | Deamer DW (2011) First Life. Berkeley CA: University of California Press. |
[12] |
Damer B, Deamer D (2020) The Hot Spring Hypothesis for an Origin of Life. Astrobiology 20: 429-452. https://doi.org/10.1089/ast.2019.2045 doi: 10.1089/ast.2019.2045
![]() |
[13] |
Holm NG, Andersson E (2005) Hydrothermal simulation experiments as a tool for studies for the origin of life on Earth and other terrestrial planets: a review. Astrobiology 5: 444-460. https://doi.org/10.1089/ast.2005.5.444 doi: 10.1089/ast.2005.5.444
![]() |
[14] |
Saladino R, Crestini C, Pino S, et al. (2012) Formamide and the origin of life. Phys Life Rev 9: 84-104. https://doi.org/10.1016/j.plrev.2011.12.002 doi: 10.1016/j.plrev.2011.12.002
![]() |
[15] |
Gaylor M, Miro P, Vlaisavljevich B, et al. (2021) Plausible Emergence and Self Assembly of a Primitive Phospholipid from Reduced Phosphorus on the Primordial Earth. Orig Life Evol Biosph 51: 185-213. https://doi.org/10.1007/s11084-021-09613-4 doi: 10.1007/s11084-021-09613-4
![]() |
[16] |
Miller SL (1953) Production of Amino Acids Under Possible Primitive Earth Conditions. Science 117: 528-529. https://doi.org/10.1126/science.117.3046.528 doi: 10.1126/science.117.3046.528
![]() |
[17] |
Markhinin EK, Podkletnov NE (1977) The phenomenon of formation of prebiological compounds in volcanic processes. Orig Life Evol Biosph 8: 225-235. https://doi.org/10.1007/BF00930684 doi: 10.1007/BF00930684
![]() |
[18] |
Yokoyama S, Koyama A, Nemoto A, et al. (2003) Amplification of diverse catalytic properties of evolving molecules in a simulated hydrothermal environment. Orig Life Evol Biosph 33: 589-595. https://doi.org/10.1023/a:1025741430748 doi: 10.1023/A:1025741430748
![]() |
[19] |
Kawamura K, Shimahashi M (2008) One-step formation of oligopeptide-like molecules from Glu and Asp in hydrothermal environments. Naturwissenschaften 95: 449-454. https://doi.org/10.1007/s00114-008-0342-7 doi: 10.1007/s00114-008-0342-7
![]() |
[20] |
Cleaves HJ, Aubrey AD, Bada JL (2009) An evaluation of critical parameters for abiotic peptide synthesis in submarine hydrothermal systems. Orig Life Evol Biosph 39: 109-126. https://doi.org/10.1007/s11084-008-9154-1 doi: 10.1007/s11084-008-9154-1
![]() |
[21] |
Deamer DW (1985) Boundary structures are formed by organic components of the Murchison carbonaceous chondrite. Nature 317: 792-794. https://doi.org/10.1038/317792a0 doi: 10.1038/317792a0
![]() |
[22] |
Martins Z, Botta O, Fogel ML, et al. (2008) Extraterrestrial nucleobases in the Murchison meteorite. Earth Plan Sci Let 270: 130-136. https://doi.org/10.1016/j.epsl.2008.03.026 doi: 10.1016/j.epsl.2008.03.026
![]() |
[23] |
Joyce G (2002) The antiquity of RNA-world evolution. Nature 418: 214-221. https://doi.org/10.1038/418214a doi: 10.1038/418214a
![]() |
[24] |
Woese CR (1987) Microbial evolution. Microbiol Rev 51: 221-270. doi: 10.1128/mr.51.2.221-271.1987
![]() |
[25] | Kompanichenko VN (2002) Life as High-Organized Form of Intensified Resistance to Destructive Processes, Fundamentals of Life, Paris: Elsevier, 111-124. |
[26] |
Kompanichenko VN (2008) Three stages of the origin-of-life process: bifurcation, stabilization and inversion. Int J Astrobiology 7: 27-46. https://doi.org/10.1017/S1473550407003953 doi: 10.1017/S1473550407003953
![]() |
[27] |
Kompanichenko VN (2012) Inversion concept of the origin of life. Orig Life Evol Biosph 42: 153-178. https://doi.org/10.1007/s11084-012-9279-0 doi: 10.1007/s11084-012-9279-0
![]() |
[28] | Kompanichenko VN (2017) Thermodynamic Inversion: Origin of Living Systems, Cham (Switzerland): Springer International Publishing. https://doi.org/10.1007/978-3-319-53512-8 |
[29] |
Kompanichenko V (2019) The Rise of A Habitable Planet: Four Required Conditions for the Origin of Life in the Universe. Geosciences 9: 92. https://doi.org/10.3390/geosciences9020092 doi: 10.3390/geosciences9020092
![]() |
[30] |
Kompanichenko V (2020) Thermodynamic Jump from Prebiotic Microsystems to Primary Living Cells. Sci 2: 14. https://doi.org/10.3390/sci2010014 doi: 10.3390/sci2010014
![]() |
[31] | Feistel R, Ebeling W (2011) Physics of Self-organization and Evolution, VCH: Wiley. |
[32] | Bukharin OV, Gintsburg AP, Romanova YM, et al. (2005) Survival mechanisms of bacteria, Moscow: Medicine. |
[33] |
El-Registan GI, Mulyukin AL, Nikolaev YA, et al. (2006) Adaptogenic functions of extracellular autoregulators of mmicroorganisms. Mikrobiologiya 75: 380-389. https://doi.org/10.1134/S0026261706040035 doi: 10.1134/S0026261706040035
![]() |
[34] | Prigogine I, Nicolis G (1977) Self-organization in Nonequilibrium Systems, New York: Wiley. |
[35] | Prigogine I, Stengers I (1984) Order out of chaos, New York: Bantam. |
[36] |
Prigogine I (1989) The philosophy of instability. Futures 21: 396-400. https://doi.org/10.1016/S0016-3287(89)80009-6 doi: 10.1016/S0016-3287(89)80009-6
![]() |
[37] | Haken H (1978) Synergetics. Berlin, New York: Springer-Verlag. |
[38] | Ebeling W, Engel A, Feistel R (1990) Physik der Evolutionsprozesse, Berlin: Akademie-Verlag. |
[39] | Kompanichenko VN (2004) Systemic approach to the origin of life. Front Perspect 13: 22-40. https://www.researchgate.net/publication/292712728 |
[40] | Selye H (1974) Stress without distress, Philadelfia & New York: JB Lippincott Company. |
[41] |
Herkovits J (2006) Evoecotoxicology: Environmental Changes and Life Features Development during the Evolutionary Process—the Record of the Past at Developmental Stages of Living Organisms. Environ Health Perspect 114. https://doi.org/10.1289/ehp.8633 doi: 10.1289/ehp.8633
![]() |
[42] | Corliss JB, Baross JA, Hoffman SE (1981) An hypothesis concerning the relationship between submarine hot springs and the origin of life on the Earth. Oceanol Acta 4: 59-69. |
[43] |
Marshall WL (1994) Hydrothermal synthesis of amino acids. Geochim Cosmochim Acta 58: 2099-2106. https://doi.org/10.1016/0016-7037(94)90288-7 doi: 10.1016/0016-7037(94)90288-7
![]() |
[44] |
Washington J (2000) The possible role of volcanic aquifers in prebiotic genesis of organic compounds and RNA. Orig Life Evol Biosph 30: 53-79. https://doi.org/10.1023/A:1006692606492 doi: 10.1023/A:1006692606492
![]() |
[45] |
Martin W, Russell JM (2007) On the origin of biochemistry at an alkaline hydrothermal vent. Philos Trans R Soc B 362: 1887-1925. https://doi.org/10.1098/rstb.2006.1881 doi: 10.1098/rstb.2006.1881
![]() |
[46] | Kolb VM (2016) Green Organic Chemistry and Its Interdisciplinary Applications, Boca Raton, CRC Press. https://doi.org/10.1201/9781315371856 |
[47] |
Van Kranendonk MJ, Baumgartner R, Djokic T, et al. (2021) Elements for the Origin of Life on Land: A Deep-Time Perspective from the Pilbara Craton of Western Australia. Astrobiology 21: 39-59. https://doi.org/10.1089/ast.2019.2107 doi: 10.1089/ast.2019.2107
![]() |
[48] |
Deamer D (2021) Where Did Life Begin? Testing Ideas in Prebiotic Analogue Conditions. Life 11: 134. https://doi.org/10.3390/life11020134 doi: 10.3390/life11020134
![]() |
[49] |
Russell MJ (2021) The "Water Problem"(sic), the Illusory Pond and Life's Submarine Emergence—A Review. Life 11: 429. https://doi.org/10.3390/life11050429 doi: 10.3390/life11050429
![]() |
[50] | Kralj P (2001) Das Thermalwasser-System des Mur-Beckens in Nordost-Slowenien. Mitteilungen zur Ingenieurgeologie und Hydrogeologie, 81: 82, RWTH Aachen, Germany. |
[51] |
Kralj P, Kralj P (2000) Thermal and mineral waters in north-eastern Slovenia. Environ Geol 39: 488-500. https://doi.org/10.1007/s002540050455 doi: 10.1007/s002540050455
![]() |
[52] | Kiryukhin AV, Lesnyikh MD, Polyakov AY. (2002) Natural hydrodynamic mode of the Mutnovsky geothermal reservoir and its connection with seismic activity. Volc Seis 1: 51-60. |
[53] |
Kompanichenko VN, Poturay VA, Shlufman KV (2015) Hydrothermal systems of Kamchatka are models of the prebiotic environment. Orig Life Evol Biosph 45: 93-103. https://doi.org/10.1007/s11084-015-9429-2 doi: 10.1007/s11084-015-9429-2
![]() |
[54] |
Ross David S, Deamer D (2016) Dry/Wet Cycling and the Thermodynamics and Kinetics of Prebiotic Polymer Synthesis. Life 6: 28. https://doi.org/10.3390/life6030028 doi: 10.3390/life6030028
![]() |
[55] |
Deamer D, Damer B, Kompanichenko V (2019) Hydrothermal Chemistry and the Origin of Cellular Life. Astrobiology 19: 1523-1537. https://doi.org/10.1089/ast.2018.1979 doi: 10.1089/ast.2018.1979
![]() |
[56] | Huxley IS (1942) Evolution: the modern synthesis, London: George Allen and Unwin. |
[57] | McCollom TM, Seewald JS (2001) A reassessment of the potential for reduction of dissolved CO2 to hydrocarbons during serpentinization of olivine. Geochim Cosmochim Acta 65: 3769-3778. Available from: https://www.elibrary.ru/item.asp?id=826854 |
[58] | Shock EL, McCollom TM, Schulte MD (1998) The emergence of metabolism from within hydrothermal systems, Thermophiles: The Keys to Molecular Evolution and the Origin of Life, Washington: Taylor and Francis, 59-76. |
[59] |
Rushdi AI, Simoneit BRT (2004) Condensation reactions and formation of amides, esters, and nitriles under hydrothermal conditions. Astrobiology 4: 211-224. https://doi.org/10.1089/153110704323175151 doi: 10.1089/153110704323175151
![]() |
[60] | Simoneit BRT (2003) Petroleum generation, extraction and migration and abiogenic synthesis in hydrothermal systems, Natural and Laboratory Simulated Thermal Geochemical Processes, Netherlands: Kluwer, 1-30. https://doi.org/10.1007/978-94-017-0111-2_1 |
[61] |
Simoneit BRT (2004) Prebiotic organic synthesis under hydrothermal conditions: an overview. Adv Space Res 33: 88-94. https://doi.org/10.1016/j.asr.2003.05.006 doi: 10.1016/j.asr.2003.05.006
![]() |
[62] | Fox S, Dose K (1975) Molecular Evolution and the Origin of Life, New York: Dekker. |
[63] |
Larralde R, Robertson M, Miller S (1995) Rates of decomposition of ribose and other sugars: implications for chemical evolution. Proc Natl Acad Sci USA 92: 8158-8160. https://doi.org/10.1073/pnas.92.18.8158 doi: 10.1073/pnas.92.18.8158
![]() |
[64] |
Vergne J, Dumas L, Decout JL, et al. (2000) Possible prebiotic catalysts formed from adenine and aldehyde. Planet Space Sci 48: 1139-1142. https://doi.org/10.1016/S0032-0633(00)00087-8 doi: 10.1016/S0032-0633(00)00087-8
![]() |
[65] |
Kazem K, Lovley DR (2003) Extending the Upper Temperature Limit for Life. Science 301: 934. https://doi.org/10.1126/science.1086823 doi: 10.1126/science.1086823
![]() |
[66] |
Varfolomeev SD (2007) Kinetic models of prebiological evolution of macromoleculer. Thermocycle as the motive force of the process. Mendeleev Commun 17: 7-9. https://doi.org/10.1016/j.mencom.2007.01.003 doi: 10.1016/j.mencom.2007.01.003
![]() |
[67] |
Osipovich DC, Barratt C, Schwartz PM (2009) Systems chemistry and Parrondo's paradox: computational models of thermal cycling. New J Chem 33: 1981-2184. https://doi.org/10.1039/b900288j doi: 10.1039/b919103h
![]() |
[68] |
Singh SV, Vishakantaiah J, Meka JK, et al. (2020) Shock Processing of Amino Acids Leading to Complex Structures—Implications to the Origin of Life. Molecules 25: 5634. https://doi.org/10.3390/molecules25235634 doi: 10.3390/molecules25235634
![]() |
[69] |
Surendra VS, Jayaram V, Muruganantham M, et al. (2021) Complex structures synthesized in shock processing of nucleobases—implications to the origins of life. Int J Astrobiology 20: 285-293. https://doi.org/10.1017/S1473550421000136 doi: 10.1017/S1473550421000136
![]() |
[70] | Mazur P (2004) Principles of cryobiology, Life in the frozen state, Boca Raton: CRC Press, 3-65. https://doi.org/10.1201/9780203647073 |
[71] |
Clarke A, Morris GJ, Fonesca F, et al. (2013) A low temperature limit for life on Earth. PLoS One 8: e66207. https://doi.org/10.1371/journal.pone.0066207 doi: 10.1371/journal.pone.0066207
![]() |
[72] |
Fonesca F, Meneghel J, Cenard S, et al. (2016) Determination of Intracellular Vitrification Temperatures for Unicellular Microorganisms under Condition Relevant for Cryopreservation. PLoS One 11: e0152939. https://doi.org/10.1371/journal.pone.0152939 doi: 10.1371/journal.pone.0152939
![]() |
[73] | Schrodinger E (1944) What is life? Cambridge University Press. |
[74] |
Frenkel-Krispin D, Minsky A (2006) Nucleoid organization and the maintenance of DNA integrity in E. coli, B. subtiles and D. radiodurans. J Struct Biol 156: 311-319. https://doi.org/10.1016/j.jsb.2006.05.014 doi: 10.1016/j.jsb.2006.05.014
![]() |
[75] |
Dadinova LA, Chsnokov IM, Kamyshinsky RA, et al. (2019) Protective Dps-DNA co-crystallization in stressed cells: an in vitro structural study by small-angle X-ray scattering and cryoelectron tomography. FEBS Letters 593: 1360-1371. https://doi.org/10.1002/1873-3468.13439 doi: 10.1002/1873-3468.13439
![]() |
[76] |
Loiko NG, Suzina NE, Soina VS, et al. (2017) Biocrysteline Structure in the Nucleoids of the Stationary and Dormant Prokaryotic Cells. Microbiology 86: 714-727. https://doi.org/10.1134/S002626171706011X doi: 10.1134/S002626171706011X
![]() |
[77] |
Parry BR, Surovtsev Ⅳ, Cabeen MT, et al. (2014) The Bacterial clytoplasm Has Glass-like Properties and Fluidized by Metabolic Activity. Cell 156: 183-194. https://doi.org/10.1016/j.cell.2013.11.028 doi: 10.1016/j.cell.2013.11.028
![]() |
[78] |
Mourão MA, Hakim JB, Schnell S (2014) Connecting the Dots: The Effects of Macromolecular Crowding on Cell Physiology. Biophys J 107: 2761-2766. https://doi.org/10.1016/j.bpj.2014.10.051 doi: 10.1016/j.bpj.2014.10.051
![]() |
[79] |
Mika JT, van der Bogaart G, Veenhoff L, et al. (2010) Molecular sieving properties of the cytoplasm of Escherichia coli and consequences of osmotic stress: Molecule diffusion and barriers in the cytoplasm. Mol Microbiol 77: 200-207. https://doi.org/10.1111/j.1365-2958.2010.07201.x doi: 10.1111/j.1365-2958.2010.07201.x
![]() |
[80] |
Antipov S, Turishchev S, Purtov Y, et al. (2017) The oligomeric forms of the Escherichia coli Dps protein depends on the availability of Iron Ions. Molecules 22: 1904. https://doi.org/10.3390/molecules22111904 doi: 10.3390/molecules22111904
![]() |
[81] | Shapiro JA, Dworkin M (1997) Bacteria as multicellular organisms, Oxford University Press. |
[82] |
Lewis K (2010) Persister cells. Annu Rev Microbiol 64: 357-372. https://doi.org/10.1146/annurev.micro.112408.134306 doi: 10.1146/annurev.micro.112408.134306
![]() |
[83] |
Podlesek Z, Butala M, Šakanovié A, et al. (2016) Antibiotic induced bacterial lysis provided a reservoir of persisters. Antonie van Leeuwenhoek 109: 523-528. https://doi.org/10.1007/s10482-016-0657-x doi: 10.1007/s10482-016-0657-x
![]() |
[84] |
Selye HA (1936) A syndrome produced by diverse nocuous agents. Nature 138: 32. https://doi.org/10.1038/138032a0 doi: 10.1038/138032a0
![]() |
[85] | Tkachenko AG (2012) Molecular mechanisms of stress responses in microorganisms. Ekaterinburg: IEGM UB RAS (In Russian). |
[86] |
Fukua WC, Winans SC, Greenberg EP (1994) Quorum sensing in bacteria: the LuxJ family of cell density—responsive transcription regulators. J Bacteriol 176: 269-275. https://doi.org/10.1128/jb.176.2.269-275.1994 doi: 10.1128/jb.176.2.269-275.1994
![]() |
[87] |
Imlay JA (2003) Pathways of oxidative damage. Annu Rev Microbiol 57: 395-418. https://doi.org/10.1146/annurev.micro.57.030502.090938 doi: 10.1146/annurev.micro.57.030502.090938
![]() |
[88] |
Imlay JA (2008) Cellular Defenses against Superoxide and Hydrogen Peroxide. Annu Rev Biochem 77: 755-776. https://doi.org/10.1146/annurev.biochem.77.061606.161055 doi: 10.1146/annurev.biochem.77.061606.161055
![]() |
[89] |
Loiko NG, Kozlova AN, Nikolaev YA, et al. (2015) Effect of stress on emergence of antibiotic-tolerant Escherichia coli cells. Mikrobiologiya 84: 595-609. https://doi.org/10.1134/S0026261715050148 doi: 10.1134/S0026261715050148
![]() |
[90] |
Mayer MP, Bukau B (2005) Hsp 70 chaperones: cellular function and molecular mechnisms. Cell Mol Life Sci 62: 670-684. https://doi.org/10.1007/s00018-004-4464-6 doi: 10.1007/s00018-004-4464-6
![]() |
[91] |
Krupyansky YF, Abdulnasyrov EG, Loiko NG, et al. (2012) Possible mechanisms of influence of hexylresorcinol on the structural-dynamic and functional properties of the lysozyme protein. Russ J Phys Chem B 6: 301-314. https://doi.org/10.1134/S1990793112020078 doi: 10.1134/S1990793112020078
![]() |
[92] | Martirosova EI, Karpekina TA, El-Registan GI (2004) Modification of enzymes by natural chemical chaperones of microorganisms. Mikrobiologiya 73: 708-715. |
[93] | Stepanenko IY, Strakhovskaya MG, Belenikina NS, et al. (2004) Protection of Saccharomyces cerevisiae with alkyloxybenzenes from oxidative and radiation damage. Mikrobiologiya 73: 204-210. |
[94] |
Azam TA, Hiraga S, Jshihama A (2000) Two types of localization of the DNA-binding protein within the Escherichia coli nucleoid. Genes Cells 5: 613-626. https://doi.org/10.1046/j.1365-2443.2000.00350.x doi: 10.1046/j.1365-2443.2000.00350.x
![]() |
[95] |
Frenkel-Krispin D, Levin-Zaidman S, Shimoni E, et al. (2001) Regulated phase Transitions of bacterial chromatin: a non-enzymatic pathway for generic DNA protection. EMBO J 20: 1184-1191. https://doi.org/10.1093/emboj/20.5.1184 doi: 10.1093/emboj/20.5.1184
![]() |
[96] |
Karas VA, Westerlaken I, Meyer AS (2013) Application of an in vitro DNA protection assay to visualize stress mediation properties of the Dps protein. J Vis Exp 75: E50390. https://doi.org/10.3791/50390 doi: 10.3791/50390
![]() |
[97] |
Grant SS, Hung DT (2013) Persistent bacterial infections, antibiotic tolerance, and oxidative stress response. Virulence 4: 273-283. https://doi.org/10.4161/viru.23987 doi: 10.4161/viru.23987
![]() |
[98] |
Mysyakina IS, Sergeeva YE, Sorokin VV, et al. (2014) Lipid and elemental composition of indicators of the physiological state of Sporangiospores in Mucor hiemalis cultures of different ages. Microbiology 83: 110-118. https://doi.org/10.1134/S0026261714020155 doi: 10.1134/S0026261714020155
![]() |
[99] |
Ignatow DK, Salina EG, Fursov MV, et al. (2015) Dormant non-culturable Mycobacterium tuberculosis retains stable low-abundant mRNA. BMC Genomics 16: 954. https://doi.org/10.1186/s12864-015-2197-6 doi: 10.1186/s12864-015-2197-6
![]() |
[100] |
Sanchez-Avila JI, Garcia Sanchez BE, Vara-Castro BM, et al. (2021) Disrtibution and origin of organic compounds in the condensates from a Mexican high-temperature hydrothermal field. Geothermics 89: 101980. https://doi.org/10.1016/j.geothermics.2020.101980 doi: 10.1016/j.geothermics.2020.101980
![]() |
[101] | Mukhin LM, Bondarev VB, Vakin EA, et al. (1979) Amino acids in hydrothermal systems in Southern Kamchatka. Doklady Acad Sci USSR 244: 974-977. |
[102] | Kompanichenko VN, Avchenko OV (2015) Thermodynamic calculations of the parameters of hydrothermal environment in the modelling of biosphere origin. Reg Probl 18: 5-13. Available from: https://readera.org/14328903 |
[103] |
Barge LM, Branscomb E, Brucato JR, et al. (2017) Thermodynamics, Disequilibrium, Evolution: Far-From-Equilibrium Geological and Chemical Considerations for Origin-Of-Life Research. Orig Life Evol Biosph 47: 39-56. https://doi.org/10.1007/s11084-016-9508-z doi: 10.1007/s11084-016-9508-z
![]() |
[104] |
Hengeveld R, Fedonkin MA (2007) Bootstrapping the energy flow in the beginning of life. Acta Biotheor 55: 181-226. https://doi.org/10.1007/s10441-007-9019-4 doi: 10.1007/s10441-007-9019-4
![]() |
[105] | Fedonkin MA (2008) Ancient biosphere: The origin, trends and events. Russ Jour Earth Sci 10: 1-9. Available from: https://www.elibrary.ru/item.asp?id=21653921. |
[106] |
Galimov EM (2006) Phenomenon of Life: between equilibrium and non-linearity. Orig Life Evol Biosph 34: 599-613. https://doi.org/10.1023/b:orig.0000043131.86328.9d doi: 10.1023/b:orig.0000043131.86328.9d
![]() |
[107] |
Kompanichenko VN (2014) Emergence of biological organization through thermodynamic inversion. Front Biosci E 6: 208-224. https://doi.org/10.2741/e703 doi: 10.2741/e703
![]() |
[108] |
Cruz-Rosas HI, Riquelme F, Ramírez-Padrón A, et al. (2020) Molecular shape as a key source of prebiotic information. J Theor Biol 499: 110316. https://doi.org/10.1016/j.jtbi.2020.110316 doi: 10.1016/j.jtbi.2020.110316
![]() |
[109] | Abel DL (2015) Primordial Prescription: the most plaguing problem of life origin science, New York: LongView Press-Academic. |
[110] |
Knauth LP, Lowe DR (2003) High Archean climatic temperature inferred from oxygen isotope geochemistry of cherts in the 3.5 Ga Swaziland Supergroup, South Africa. GSA Bull 115: 566-580. https://doi.org/10.1130/0016-7606(2003)115 < 0566:HACTIF > 2.0.CO; 2 doi: 10.1130/0016-7606(2003)115 < 0566:HACTIF > 2.0.CO; 2
![]() |
1. | Vladimir Kompanichenko, Oleg Kotsyurbenko, Role of Stress in the Origin of Life, 2022, 12, 2075-1729, 1930, 10.3390/life12111930 | |
2. | Oleg R. Kotsyurbenko, Vladimir N. Kompanichenko, Anatoli V. Brouchkov, Yuliya Y. Khrunyk, Sergey P. Karlov, Vladimir V. Sorokin, Dmitry A. Skladnev, Different Scenarios for the Origin and the Subsequent Succession of a Hypothetical Microbial Community in the Cloud Layer of Venus, 2024, 24, 1531-1074, 423, 10.1089/ast.2022.0117 |