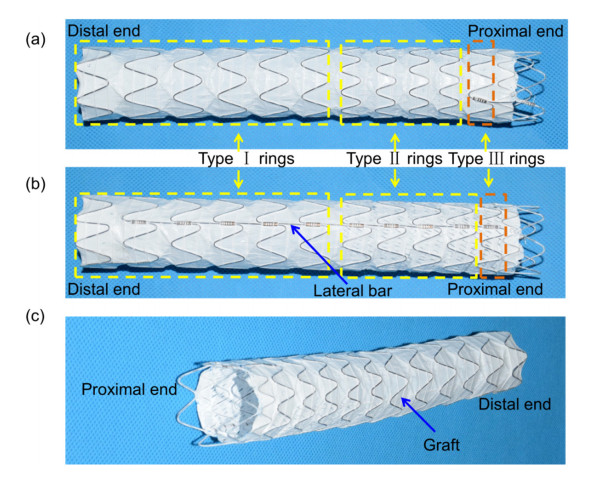
We consider the following Schrödinger system
{−Δuj=k∑i=1βij|ui|3|uj|uj+λj|uj|q−2uj, inΩ,uj=0on∂Ω,j=1,⋯,k
where Ω⊂R3 is a bounded domain with smooth boundary. Assume 5<q<6,λj>0,βjj>0,j=1,⋯,k, βij=βji,i≠j,i,j=1,⋯,k. Note that the nonlinear coupling terms are of critical Sobolev growth in dimension 3. We prove that under an additional condition on the coupling matrix the problem has infinitely many sign-changing solutions. The result is obtained by combining the method of invariant sets of descending flow with the approach of using approximation of systems of subcritical growth.
Citation: Changmu Chu, Jiaquan Liu, Zhi-Qiang Wang. Sign-changing solutions for Schrödinger system with critical growth[J]. Electronic Research Archive, 2022, 30(1): 242-256. doi: 10.3934/era.2022013
[1] | Yonca Erdem Demirtaş, Neslihan Fidan Keçeci . The efficiency of private pension companies using dynamic data envelopment analysis. Quantitative Finance and Economics, 2020, 4(2): 204-219. doi: 10.3934/QFE.2020009 |
[2] | Lei Mao, Yan Zhang . Robust optimal excess-of-loss reinsurance and investment problem with p-thinning dependent risks under CEV model. Quantitative Finance and Economics, 2021, 5(1): 134-162. doi: 10.3934/QFE.2021007 |
[3] | Hongxuan Huang, Zhengjun Zhang . An intrinsic robust rank-one-approximation approach for currency portfolio optimization. Quantitative Finance and Economics, 2018, 2(1): 160-189. doi: 10.3934/QFE.2018.1.160 |
[4] | Anna Timonina-Farkas . COVID-19: data-driven dynamic asset allocation in times of pandemic. Quantitative Finance and Economics, 2021, 5(2): 198-227. doi: 10.3934/QFE.2021009 |
[5] | Gülserim Özcan . The amplification of the New Keynesian models and robust optimal monetary policy. Quantitative Finance and Economics, 2020, 4(1): 36-65. doi: 10.3934/QFE.2020003 |
[6] | Jorge A. Chan-Lau . Systemic centrality and systemic communities in financial networks. Quantitative Finance and Economics, 2018, 2(2): 468-496. doi: 10.3934/QFE.2018.2.468 |
[7] | Thomas C. Chiang . Stock returns and inflation expectations: Evidence from 20 major countries. Quantitative Finance and Economics, 2023, 7(4): 538-568. doi: 10.3934/QFE.2023027 |
[8] | Vladimir Donskoy . BOMD: Building Optimization Models from Data (Neural Networks based Approach). Quantitative Finance and Economics, 2019, 3(4): 608-623. doi: 10.3934/QFE.2019.4.608 |
[9] | AiMin Yan, Hao Ma, Dandan Zhu, Julan Xie . Digital transformation and corporate resilience: Evidence from China during the COVID-19 pandemic. Quantitative Finance and Economics, 2024, 8(4): 779-814. doi: 10.3934/QFE.2024030 |
[10] | Salvatore Scognamiglio . Longevity risk analysis: applications to the Italian regional data. Quantitative Finance and Economics, 2022, 6(1): 138-157. doi: 10.3934/QFE.2022006 |
We consider the following Schrödinger system
{−Δuj=k∑i=1βij|ui|3|uj|uj+λj|uj|q−2uj, inΩ,uj=0on∂Ω,j=1,⋯,k
where Ω⊂R3 is a bounded domain with smooth boundary. Assume 5<q<6,λj>0,βjj>0,j=1,⋯,k, βij=βji,i≠j,i,j=1,⋯,k. Note that the nonlinear coupling terms are of critical Sobolev growth in dimension 3. We prove that under an additional condition on the coupling matrix the problem has infinitely many sign-changing solutions. The result is obtained by combining the method of invariant sets of descending flow with the approach of using approximation of systems of subcritical growth.
Thoracic endovascular aortic repair (TEVAR) is a minimally invasive procedure for the aortic arch diseases; it reduces the operative morbidity and mortality compared with the traditional open surgical repair procedure [1,2,3]. However, the procedure mainly benefits descending aorta diseases. It still remains significant challenges for the aortic arch diseases due to the complex morphological structures and severely tortuous aortic arch [4]. Maintaining the blood perfusion in branches and good sealing effect are the priorities for the application of TEVAR at the aortic arch [5]. More recently, a hybrid operative procedure and chimney graft technique have been proposed to preserve aortic lateral branches' perfusion [6,7]. However, an inadequate seal between the SGs and the vessel is still a challenging problem.
Flexibility is one of the critical features of an SG for clinical applications [8] and determines the SG's ability to fit the vessels [9]. If the flexibility is insufficient, the SG will be easily twisted when guided through tortuous arteries, causing complications [10,11,12,13]. On the contrary, good flexibility enables the expanded stent to follow the vessel's contour and reduce distortion at the stent‒vessel interface [12]. Accordingly, improved flexibility will extend the application of stents in a wide range of anatomical vascular morphology [13,14]. Endoleaks might exist if the expanded device does not adapt to the artery properly due to the lack of flexibility [15,16,17]. Therefore, the improved flexibility of SGs can also enhance the sealing effect, minimizing endoleak, and other complication risks [2].
Numerical methods have been applied in investigating the biomechanical behaviors of SGs for the low-cost compared with experimental tests [18,19,20]. Complex biomechanical environments can be ideally simulated in vivo and in vitro to assess the device performance [21,22,23]. Flexibility is a crucial performance of SGs in EVAR, with a close relationship with complications [2,24]. The stent flexibility is influenced significantly by the design of SGs. Spiral and circular stents are more flexible than the Z-shaped ones [12,15]. Stent structures also affect the device flexibility at both expanded and unexpanded states [9,14]. The wire rings connected by the point symmetrical links can improve stent flexibility [25]. The multi-linked stent possessed the best longitudinal flexibility, and the crown stent was the stiffest in a series of slotted tube designs [12]. Apart from the shape and structure of SGs, other geometric parameters also influence flexibility. A larger strut radius and smaller strut width can make the stent more flexible [26,27,28]. A decrease in flexibility of braided monofilament stent with increased wire diameter and strut number has also been confirmed [29]. In addition, the graft properties also influence the device directly; a highly flexible stent has been developed, assembled with ultra-thin silicone covering [30]. These mainly concentrate at the abdominal aorta or the carotid artery SGs; very few studies have focused on the thoracic aorta SGs. The geometric parameters' influential degrees on the SG flexibility have not been studied yet. On the other hand, factors or even one single factor have partially been investigated; however, the major influences of geometric parameters on the device flexibility are not clear yet. The durability of SGs induced by both the stent and fabric fracture should be focused on during the analyses. Fabric tear might be more frequent with Z-shaped SGs. Although it is very rare in the clinical reports about the fabric tear [11,31], complications induced by the fabric tear have been reported [32]. However, the durability of SGs influenced by the geometrical parameters has not been studied.
Therefore, this work aimed to investigate the thoracic aorta SGs in terms of the influence of geometric parameters on flexibility and durability via FE analyses. First, 16 FE models were established with an orthogonal design with five factors and four levels. Then, the influences of a single factor and the geometric parameters' influences on the device flexibility were determined. Lastly, SGs' structural durability during bending was estimated. The influence of the parameters was also examined in the design process.
FEA can be used to carry out repeated tests with a lower cost, find the most vulnerable point in the model, and optimize it. As one of its merits, the FEA can simulate the effect before surgery, which cannot be tried in clinical practice. The finite element models of stent-grafts with different geometrical parameters can be modeled easily and quickly and can be used to reveal the stress and strain distributions of each position.
A commercially available thoracic aorta SG (Lifetech, Shenzhen, Guangdong Province, China) was selected as the basic design in the current study (Figures 1 and 2). The SG consists of six Type Ⅰ rings near the distal end, four Type Ⅱ rings located in the middle of the SG, and one Type Ⅲ ring that closes to the right of Type Ⅱ rings. The detailed dimensions of the ring Types are summarized and presented in Table 1 and Figure 3. In addition, one ring that contains twelve struts measuring 7 mm in height and one bell-shaped ring is set at the proximal end to improve the anchoring ability of SG within the vessels. In the commercial SG, all the struts have a radius of 1.2 mm, and all the metal wires have a wire diameter of 0.55 mm. All the rings are soldered to the lateral bars (Figure 1b). In the FE model, there are three Type Ⅰ rings, four Type Ⅱ rings, and one Type Ⅲ ring. All the rings were connected by the straight lateral bar. The bare metal stent was fully covered with an 0.1-mm-thick and 150-mm-long graft (Figure 2c). The 3D geometric model was established in Solidworks (Dassault Systems Corp, Massachusetts, USA).
Ring type | Ring number | Strut number(each ring) | Strut height(mm) | Strut radius(mm) |
Type Ⅰ | 3 | 7 | 15 | 1.2 |
Type Ⅱ | 4 | 4, 5 | 14, 9 | 1.2 |
Type Ⅲ | 1 | 9 | 12 | 1.2 |
Most of the previous stent flexibility studies have focused on the influence of single or double factors [9,28,29]. The influence of multiple factors has rarely been studied, and the important ranking of the parameters has not been investigated. In this study, five geometric parameters, including four from the bare stent (strut height, strut number, strut radius, and wire diameter) and one from the graft (thickness), were investigated in terms of SG flexibility and durability at the same time (Figure 3). Orthogonal analysis of five factors and four levels was designed to determine the effects of geometric parameters on SG's performance simultaneously, using an efficient and inexpensive method. Hence, 16 specimens were analyzed. Table 2 lists the geometric features of every specimen. Specimen 1 (sp1) was defined as the original model. A to E represent the geometric parameters in terms of strut height, strut number, strut radius, wire diameter, and graft thickness, marked in Figure 3. The numbers 1 to 4 denote the factor levels. Since the strut height and strut number in the three types of the rings were different, the calculations would increase enormously if the real heights and strut numbers of every ring type were defined as the factors. Therefore, factors A and B of all the rings were described by their variation (Delta value) compared with the original model (Table 3). For instance, the 2nd level value of factor A meant that all the strut heights were 2 mm shorter than sp1. The 2nd level of factor B meant that there were two fewer struts in all the rings than sp1. Table 2 summarizes the values of Ai to Ei (i = 1, 2, 3, 4). For example, compared with sp1, sp8 has two fewer struts in each ring, and its ring strut height is 2 mm shorter. Besides, sp8 had a strut radius of 0.8 mm, a wire diameter of 0.65 mm, and was covered with an 0.1 mm-thick graft.
Specimen number | Factors | Performances | |||||||
A | B | C | D | E | Global flexibility | Local flexibility | Durability | ||
1 | 1 | 1 | 1 | 1 | 1 | RM | SV | LS | CS |
2 | 1 | 2 | 2 | 2 | 2 | RM | SV | LS | CS |
3 | 1 | 3 | 3 | 3 | 3 | RM | SV | LS | CS |
4 | 1 | 4 | 4 | 4 | 4 | RM | SV | LS | CS |
5 | 2 | 1 | 2 | 3 | 4 | RM | SV | LS | CS |
6 | 2 | 2 | 1 | 4 | 3 | RM | SV | LS | CS |
7 | 2 | 3 | 4 | 1 | 2 | RM | SV | LS | CS |
8 | 2 | 4 | 3 | 2 | 1 | RM | SV | LS | CS |
9 | 3 | 1 | 3 | 4 | 2 | RM | SV | LS | CS |
10 | 3 | 2 | 4 | 3 | 1 | RM | SV | LS | CS |
11 | 3 | 3 | 1 | 2 | 4 | RM | SV | LS | CS |
12 | 3 | 4 | 2 | 1 | 3 | RM | SV | LS | CS |
13 | 4 | 1 | 4 | 2 | 3 | RM | SV | LS | CS |
14 | 4 | 2 | 3 | 1 | 4 | RM | SV | LS | CS |
15 | 4 | 3 | 2 | 4 | 1 | RM | SV | LS | CS |
16 | 4 | 4 | 1 | 3 | 2 | RM | SV | LS | CS |
K1 | KA1 | KB1 | KC1 | KD1 | KE1 | ||||
K2 | KA2 | KB2 | KC2 | KD2 | KE2 | ||||
K3 | KA3 | KB3 | KC3 | KD3 | KE3 | ||||
K4 | KA4 | KB4 | KC4 | KD4 | KE4 | ||||
R | KmaxAi−KminAi | KmaxBi−KminBi | KmaxCi−KminCi | KmaxDi−KminDi | KmaxEi−KminEi |
![]() |
A | B | C | D | E |
1 | 0 | 0 | 1.2 mm | 0.55 mm | 0.1 mm |
2 | 2 | 2 | 1.5 mm | 0.65 mm | 0.12 mm |
3 | −2 | 4 | 0.8 mm | 0.45 mm | 0.08 mm |
4 | −4 | −2 | 0.6 mm | 0.35 mm | 0.06 mm |
The maximum reaction moment (RM), the spacing variation of the Type Ⅱ rings, and both the circumferential strain (CS) and longitudinal strain (LS) in all the specimens can be obtained after the analyses are completed. The Ki (i = 1, 2, 3, 4) in the orthogonal table is used to analyze each factor's effect. They are calculated by summing the values obtained from the same factor under the same level i among all the models. For instance, when KB1 is used to analyze the influence on factor B's global flexibility under the 1st level, it is calculated by summing up the RMs obtained in sp1, sp5, sp9, and sp13. After confirming all the Ki values, R can be used to reflect all the factors' influence degree, where R is obtained from the difference of the maximum and minimum Ki. A higher R-value means that the factor has a more remarkable influence on the flexibility or durability.
Due to the remarkable super-elasticity, high flexibility, and shape memory effect [23], the self-expanding nitinol stent has been a popular choice in the TEVAR. The nitinol stent can be crimped easily at a low temperature, returning to its predesigned profile after being released from the catheter at the body temperature. The expanded polytetrafluoroethylene (e-PTFE) is a biocompatible, highly nonreactive, and nontoxic polymer. Currently, it is one of the most popular graft materials [21,31]. In this study, a nitinol stent covered with e-PTFE graft was employed, and the main material properties are presented in Table 4. The nitinol material was modeled with a user material subroutine in ABAQUS 6.14 (Simulia, Providence, RI, USA) [23].
Nitinol | |
Austenite Young's modulus EA (MPa) | 650000 |
Austenite Poisson's ratio μA | 0.33 |
Martensite Young's modulus EA (MPa) | 40000 |
Martensite Poisson's ratio μM | 0.33 |
Transformation strain εL | 0.041 |
Loading (δσ/δT)L(MPaT−1) | 6.7 |
Start of transformation loading σSL(MPa) | 440 |
End of transformation loading σEL(MPa) | 540 |
Reference temperature T0 (℃) | 22 |
Unloading (δσ/δT)U(MPaT−1) | 6.7 |
Start of transformation loading σSU(MPa) | 250 |
End of transformation loading σEU(MPa) | 140 |
Strain limit εmax | 12% |
e-PTFE graft | |
Young's modulus E (MPa) | 55.2 |
Poisson's ratio μ | 0.46 |
Ultimate strain εR | 20% |
The FE analyses were undertaken in ABAQUS. The boundary and loading conditions during the analyses are presented in Figure 4a. Both ends of the device were considered as rigid bodies controlled by reference points (RP). The rotations about the x-axis and y-axis were locked. The translations along x and z axes were limited at the reference points. During the analysis, the constraint along the y-axis was released, and opposite rotations (α/2) were applied on the reference points about the z-axis (Figure 4a). To ensure SG's adequate flexibility in the tortuous vessel, the bending angle α here was 90°, which was much higher than the average angle of the aortic arch [33]. The "tie constraint" was employed in ABAQUS to mimic the suture between the metal and graft to prevent sliding and separation during the analyses. The self-contact algorithm was enabled to prevent the self-penetration of the components. As the FE analyses involved high nonlinearities, a dynamic explicit algorithm was employed to prevent non-convergence. The dynamic effect was limited to < 5% of static effects during the analyses [34].
The metal stent was meshed with the 2-nodes beam element (B31), and the graft was meshed with the S4 shell element (Figure 4b). The original model was used to verify the convergence of meshes with five element sizes. The detailed verification is presented in the Supplementary section. Finally, an element size of 0.6 mm was selected because it had acceptable accuracy and lower computing cost. There were approximately 3300 elements in the stent and 45000 elements in the graft.
In this study, the variable geometric parameters' influence on the SG's flexibility and durability was studied. The flexibility study included both global flexibility and local flexibility. A durability study was conducted for both the stent and graft, respectively.
A few assessment criteria have been proposed and applied to the flexibility analyses of SGs with different shapes [10,15]. Some parts of the criteria were adopted and extended to assess the devices' global and local flexibility in this study. The global flexibility reflected the deformability of the stent under the moment and was defined in this study as:
δ=1/RM |
where RM was the reaction moment. The higher value of δ indicated better global flexibility. Finally, the global flexibility was assessed by the maximum reaction moment during bending. The global flexibility can be used to reflect the ease of SG transportation within tortuous arteries. After the SG is released within the thoracic aorta, Type Ⅱ rings are always placed at the aortic arch; hence, adequate local flexibility is required to ensure the sealing effects between the SG and aortic arch.
Local flexibility was first proposed in this study and defined as the spacing variation (SV) along the y-axis between the nodes A and B (Figure 4c). The SV was calculated by the equation:
SV=1−L/L0 |
where L0 and L are the spaces between the nodes under the un-deformed and deformed states, respectively. A greater SV change reflects better local flexibility.
The durability of the stent and graft was considered respectively during the bending process. The maximum strains of the graft were obtained at both the longitudinal (Figure 5, Z-axial) and circumferential (Figure 5, T-axial) directions during the analyses. Therefore, a cylindrical coordinate system was created to extract the results. The graft's ultimate strains at the longitudinal and circumferential directions were set at 20% equally, and the strain limit of the nitinol alloy was 12% (Table 4).
All the device deformations after bending could be roughly divided into two categories in terms of the deformation shapes: "V" and "U" (Figure 6). The "V" shape was observed in sp6, sp7, sp9, sp10, and sp15, while the "U" shape was seen in the other specimens. In the "V" shape, the graft was folded severely in the middle, where the displacements were also obviously higher than the other positions. Slight wrinkles occurred at the distal and proximal ends of V-shaped specimens. By contrast, the relatively uniformly distributed wrinkles and displacement were found in the "U" shape. The maximum displacements in U-shaped specimens were less than those in V-shaped specimens. Stretching was also observed in the transverse direction of V-shaped specimens. Additionally, distinct wave shapes associated with the metal wire were observed in the interior aspects of sp4, sp8, sp12, and sp16, which had the least strut numbers. This phenomenon did not occur in other specimens.
Figure 7a summarizes the maximum RM values during the bending process. The RM reflected the device's global flexibility directly, and higher RM indicated lower flexibility. In all the models, sp2, sp7, sp8, sp9, sp10, and sp16 exhibited higher values than the sp1, and the other models exhibited lower values. Approximate values, which meant similar global flexibility, were observed in sp4 and sp14; sp5, sp11, sp12, and sp13; and sp6 and sp15, respectively. sp14 and sp4 needed the lowest RM to achieve the predefined shapes while sp2 and sp7 were the most difficult to be bent. The RM of sp7 was more than twice of sp1 and four times of sp14. Hence, sp7 exhibited the lowest flexibility, while sp14 and sp4 were the most flexible models.
Figure 7b-e shows the individual geometric parameters' influences on the maximum RM. The Ki in Figures 7b and 7f monotonically increased in association with increased strut height and graft thickness. Although the Ki fluctuated in Figures 7c and 7e, it tended to be positively related to the increased value of geometric parameters, as shown in Figures 7b and 7f. The strut radius was the only factor to influence Ki inversely. In addition, there were no significant differences in Ki in Figures 7d and 7e, when the strut radius reached 0.8 mm, or the wire diameter reached 0.45 mm. The ranges of Ki obtained from all the factors are presented in Figure 7g. The Ki of graft thickness ranged more widely than those of the other factors, which meant that the graft thickness was the most crucial factor. The strut height was the second most crucial factor in influencing RM. Approximate incidence was obtained from the verified strut number and wire diameter, like from the strut height. The strut radius exerted the slightest effect on RM. Obviously, the importance of factors on RM or global flexibility was E > A > B > D > C in descending order.
The device's local flexibility was assessed by the SV of Type Ⅱ rings, as depicted in Figure 8. The SV in sp9 was the highest in all models, and it was significantly higher than sp1. Specimen16 exhibited the lowest variation, but it was not much lower than sp1, sp11, and sp13; it was close to sp2, sp12, and sp14. In addition, the V-shaped models mentioned in section 3.1 exhibited greater SVs than the other specimens (Figure 8a), and there were no significant differences between them. It presented better local flexibility in V-shaped models than the U-shaped models. In addition to the V-shaped specimens, sp3, sp4, sp5, and sp8 also exhibited higher SV than the original specimen. The SV of sp4 was the highest (approximately 20% higher than sp1) in all the U-shaped models, indicating that sp4 had the best local flexibility.
Figure 8b-f lists the influences of a single factor on the local flexibility. Overall, the strut height, strut number, and graft thickness positively impacted the local flexibility; however, there were fluctuations during the process. The Ki decreased rapidly in association with the increased strut radius and wire diameter. The Ki tended to be stable when the strut radius reached 1.2 mm. However, the Ki obtained from the wire diameter continued to decrease. Figure 8g shows the Ki ranges obtained from all the factors. The graft thickness was the weakest factor in influencing local flexibility, contrary to the wire diameter, which significantly influenced local flexibility. There were no significant differences between the ranges obtained from the strut height, strut number, and strut radius. Finally, the local flexibility's critical ranking was: D > B > A > C > E.
Figures 9a and 9b separately present the maximum strains in the longitudinal and circumferential directions under a cylindrical coordinate system. Only the circumferential strain of sp7 exceeded the strain limitεR, and the others all stayed in a safe range in both directions. The graft of sp14 exhibited the highest durability for its lowest strains in both directions among all the models. The maximum strain of the metal stent in sp6 was the highest during the bending process. Nevertheless, it was much less than the limitεlim. The durability of metal stents was not discussed in this study.
Figure 10a-e shows the influences on the graft durability of single factors. The factors positively impacted the circumferential and maximum longitudinal strains, as shown in Figures 10a and 10e. However, the reverse phenomenon occurred in Figure 10d. The maximum strains in both directions decreased with an increase in the wire diameter, indicating an increase in durability. Meanwhile, the maximum circumferential strain increased monotonically with an increase in the strut number while the maximum longitudinal strain fluctuated smoothly within the range (Figure 10b). When the strut radius increased from 0.6 to 0.8 mm, the maximum strains in both directions decreased simultaneously. Then, the maximum circumferential strain changed slightly, and the maximum longitudinal strain increased linearly with the increased strut radius. Figure 10f summarizes the importance of geometric parameters on device durability. The graft thickness exerted the most significant influence on the maximum longitudinal strain, and the strut height influenced the circumferential durability significantly. The factors influencing the circumferential and longitudinal durability in descending order were as follows: A > E > B > C > D and E > A > D > C > B, respectively.
Improved flexibility might extend the application of SGs in the wide range of anatomical vascular morphology [14,15]. It has been verified that device flexibility is influenced by geometric parameters of the metal stent and graft [29,30]. However, influences on SGs' flexibility, mainly from one or few parameters, had been studied. Here, the thoracic aorta SGs were designed and optimized in terms of the influence of comprehensive geometric parameters on flexibility. The influences of each parameter were obtained, and the important ranking of all the impacting factors was examined. At the same time, the influences on device durability during the bending process were also investigated. Our study's results indicated that these geometric parameters exerted different influences on flexibility and durability, which provides the strategy of designing the thoracic aortic SGs, especially for the thoracic aortic arch diseases.
The thoracic aorta SG selected in this study had a special structure that contained three types of wire rings connected by a lateral bar. Due to the lower bending stiffness, the device was more flexible at the Type Ⅱ wire rings. Therefore, the SV of Type Ⅱ rings was proposed as local flexibility, which was analyzed in association with global flexibility.
Here, the significant effect on global flexibility mainly came from the graft properties. Although the other parameters were different among the specimens, a common thread occurred in sp2, sp7, sp9, and sp16; they were covered with the thickest graft and had much higher RM than the other models (Figure 7a). A similar phenomenon also occurred in sp14 and sp4, covered with the thinnest graft, and with the lowest RM. In addition, the graft thickness was confirmed as the most influential factor on global flexibility in terms of range analyses, and its effect surpassed the other factors (Figure 7g). For all metal stent parameters, except the strut radius, their increase brought about lower global flexibility. Partial parameters have been confirmed or reported in previous studies [26,29]. Among all the models, the sp14 exhibited the most favorable parameters from the graft and the metal stent on improving global flexibility, and it becomes the most flexible device.
Contrary to sp4, sp7 gathered the most impact factors that would reduce global flexibility except for the wire diameter. Although the wire diameter was not the weightiest factor on global flexibility (Figure 7g), the original size of the wire diameter (0.55 mm) induced a higher RM (Figure 7e). Thus, sp7 exhibited the highest RM corresponding with the worst global flexibility.
Distinct "V" shapes and transverse stretches were observed in sp6, sp7, sp9, sp10, and sp15 (Figure 6a), representing drastic shape changes of the cross-section, which were adopted as an assessment criterion for device flexibility [10]. The higher SVs in Figure 8a demonstrated their better local flexibility. The curves had the same trend as in Figures 7b, 7c, 7d, and 7f, and Figure 8b-f; however, they represented the opposite effects on global and local flexibility. The wire diameter was the unique factor that had the same effect on both global and local flexibility. Therefore, it was difficult to achieve the best global and local flexibility in the same specimen. For instance, sp7 had the weakest global flexibility, but it also had almost the best local flexibility in all the models; sp4 had the optimum factor (thinnest graft and the most delicate wire) among all the models for improving global and local flexibility, with better global and local flexibility synchronously. Unlike the global flexibility analyses, the most significant influencing factor was wire diameter, and the weakest factor was the graft thickness in influencing local flexibility (Figure 8g). The importance of strut numbers did not exhibit significant differences in influencing global and local flexibility.
In the current study, the influences of geometrical parameters on the durability of SGs were also summarized, which provides a strategy for designing thoracic aortic SGs. Variations in geometric parameters of SG induced different folding behaviors of graft, as shown in Figure 6. The strains were related to the folding behavior. In other words, the durability of the graft would be influenced by geometric parameters. The maximum circumferential strain in sp7 demonstrated that graft durability should not be neglected. Figure 10f also shows that the most critical impacts on circumferential and longitudinal strains were the strut height and graft thickness, respectively. Variations in the two factors also have the same significant effect on global flexibility (Figure 7g). Therefore, decreased strut height and graft thickness properly leads to increased global flexibility and graft durability.
In summary, our results indicated that these geometric parameters exerted different influences on flexibility and durability. An SG with better global flexibility can be easily transmitted in the tortuous vessels, and a device with better local flexibility is more applicable for the thoracic aortic arch disease to avoid endoleak.
This work was supported by the China-Singapore Joint Research Program (No. 2016YFE0117200).
The authors declare that they have no competing interests.
[1] | T. Bartsch, Z.-Q. Wang, Note on ground states of nonlinear Schrödinger systems, Partial Differ. Equ., 3 (2006), 200–207. |
[2] |
Z. Chen, W. Zou, On an elliptic problem with critical exponent and Hardy potential, J. Differ. Equ., 252 (2012), 969–987. https://doi.org/10.1016/j.jde.2011.09.042 doi: 10.1016/j.jde.2011.09.042
![]() |
[3] |
Z. Chen, W. Zou, Ground states for a system of Schrödinger equations with critical exponent, J. Funct. Anal., 262 (2012), 3091–3107. https://doi.org/10.1016/j.jfa.2012.01.001 doi: 10.1016/j.jfa.2012.01.001
![]() |
[4] |
Z. Chen, C.-S. Lin, W. Zou, Infinitely many sign-changing and semi-nodal solutions for a nonlinear Schrödinger system, Ann. Sc. Norm. Super. Pisa Cl. Sci., 15 (2016), 859–897. https://doi.org/10.2422/20362145.201401_002 doi: 10.2422/20362145.201401_002
![]() |
[5] |
T.-C. Lin, J. Wei, Ground state of N coupled nonlinear Schrödinger equations in Rn,n≤3, Comm. Math. Phys. 255 (2005), 629–653. https://doi.org/10.1007/s002200051313x doi: 10.1007/s002200051313x
![]() |
[6] |
Z. Liu, Z.-Q. Wang, Multiple bound states of nonlinear Schrödinger systems, Comm. Math. Phys., 282 (2008), 721–731. https://doi.org/10.1007/s002200080546x doi: 10.1007/s002200080546x
![]() |
[7] |
Z. Liu, Z.-Q. Wang, Ground States and Bound States of a Nonlinear Schrödinger System, Adv. Nonlinear Stud., 10 (2010), 175–193. https://doi.org/10.1515/ans20100109 doi: 10.1515/ans20100109
![]() |
[8] |
J. Liu, X. Liu, Z.-Q. Wang, Multiple mixed states of nodal solutions for nonlinear Schrödinger systems, Calc. Var. Partial Differ. Equ., 52 (2015), 565–586. https://doi.org/10.1007/s005260140724y doi: 10.1007/s005260140724y
![]() |
[9] |
J. Liu, X. Liu, Z.-Q. Wang, Sign-changing solutions for coupled nonlinear Schrödinger equations with critical growth, J. Differ. Equ., 261 (2016), 7194–7236. https://doi.org/10.1016/j.jde.2016.09.018 doi: 10.1016/j.jde.2016.09.018
![]() |
[10] | G. Devillanova, S. Solimini, Concentrations estimates and multiple solutions to elliptic problems at critical growth, Adv. Differ. Equ., 7 (2002), 1257–1280. |
[11] |
D. Cao, S. Peng, S. Yan, Infinitely many solutions for p-Laplacian equation involving critical Sobolev growth, J. Funct. Anal., 262 (2012), 2861–2902. https://doi.org/10.1016/j.jfa.2012.01.006 doi: 10.1016/j.jfa.2012.01.006
![]() |
[12] |
Z. Chen, C.-S. Lin, W. Zou, Sign-changing solutions and phase separation for an elliptic system with critical exponent, Comm. Partial Differ. Equ., 39 (2014), 1827–1859. https://doi.org/10.1080/03605302.2014.908391 doi: 10.1080/03605302.2014.908391
![]() |
[13] |
J. Zhao, X. Liu, J. Liu, p-Laplacian equations in RN with finite potential via truncation method, the critical case, J. Math. Anal. Appl., 455 (2017), 58–88. https://doi.org/10.1016/j.jmaa.2017.03.085 doi: 10.1016/j.jmaa.2017.03.085
![]() |
[14] |
T. Bartsch, Critical point theory on partially ordered Hilbert spaces, J. Funct. Anal., 186 (2001), 117–152. https://doi.org/10.1006/jfan.2001.3789 doi: 10.1006/jfan.2001.3789
![]() |
[15] |
T. Bartsch, Z.-Q. Wang, On the existence of sign changing solutions for semilinear Dirichlet problems, Topol. Methods Nonlinear Anal., 7 (1996), 115–131. https://doi.org/10.12775/TMNA.1996.005 doi: 10.12775/TMNA.1996.005
![]() |
[16] |
T. Bartsch, K.-C. Chang, Z.-Q. Wang, On the Morse indices of sign changing solutions of nonlinear elliptic problems, Math. Z., 233 (2000), 655–677. https://doi.org/10.1007/s002090050492 doi: 10.1007/s002090050492
![]() |
[17] |
T. Bartsch, Z. Liu, T. Weth, Sign changing solutions of superlinear Schrödinger equations, Commun. Partial Differ. Equ., 29 (2004), 25–42. https://doi.org/10.1081/PDE-120028842 doi: 10.1081/PDE-120028842
![]() |
[18] |
T. Bartsch, Z. Liu, T. Weth, Nodal solutions of a p-Laplacian equation, Proc. Lond. Math. Soc., 91 (2005), 129–152. https://doi.org/10.1112/S0024611504015187 doi: 10.1112/S0024611504015187
![]() |
[19] |
S. Li, Z.-Q. Wang, Ljusternik-Schnirelman theory in partially ordered Hilbert spaces, Trans. Amer. Math. Soc., 354 (2002), 3207–3227. https://doi.org/10.1090/S0002994702030313 doi: 10.1090/S0002994702030313
![]() |
[20] |
Z. Liu, J. Sun, Invariant sets of descending flow in critical point theory with applications to nonlinear differential equations, J. Differ.l Equ., 172 (2001), 257–299. https://doi.org/10.1006/jdeq.2000.3867 doi: 10.1006/jdeq.2000.3867
![]() |
[21] | P. Rabinowitz, Minimax Methods in Critical Point Theory with Applications to Differential Equations. CBMS Regional Conf. Ser. in Math., vol. 65. American Mathematical Society, Providence, 1986. https://doi.org/10.1090/cbms/065 |
[22] | K. Tintarev, K.-H. Fieseler, Concentration compactness. Functional-analytic grounds and applications. Imperial College Press, London, 2007. https://doi.org/10.1142/p456 |
1. | Pejman Peykani, Emran Mohammadi, Reza Farzipoor Saen, Seyed Jafar Sadjadi, Mohsen Rostamy-Malkhalifeh, Data envelopment analysis and robust optimization: A review, 2020, 37, 02664720, e12534, 10.1111/exsy.12534 | |
2. | Peirong Chen, Ruhe Xie, Mingxuan Lu, “Resource Conservation” or “Environmental Friendliness”: How do Urban Clusters Affect Total-Factor Ecological Performance in China?, 2020, 17, 1660-4601, 4361, 10.3390/ijerph17124361 | |
3. | Emmanuel Kwasi Mensah, Robust data envelopment analysis via ellipsoidal uncertainty sets with application to the Italian banking industry, 2020, 43, 1593-8883, 491, 10.1007/s10203-020-00299-3 | |
4. | Mohammad Izadikhah, Elnaz Azadi, Majid Azadi, Reza Farzipoor Saen, Mehdi Toloo, Developing a new chance constrained NDEA model to measure performance of sustainable supply chains, 2020, 0254-5330, 10.1007/s10479-020-03765-8 | |
5. | Sa Xu, Zejun Li, The Impact of Innovation Activities, Foreign Direct Investment on Improved Green Productivity: Evidence From Developing Countries, 2021, 9, 2296-665X, 10.3389/fenvs.2021.635261 | |
6. | Maziar Salahi, Mehdi Toloo, Narges Torabi, A new robust optimization approach to common weights formulation in DEA, 2020, 0160-5682, 1, 10.1080/01605682.2020.1718016 | |
7. | Mengxin Wang, Yanling Li, Gaoke Liao, Spatial Spillover and Interaction Between High-Tech Industrial Agglomeration and Urban Ecological Efficiency, 2022, 10, 2296-665X, 10.3389/fenvs.2022.829851 | |
8. | Rita Shakouri, Maziar Salahi, Zeljko Stevic, Stochastic P-Robust Approach to a Centralized Two-Stage DEA System with Resource Waste, 2022, 2022, 1687-9155, 1, 10.1155/2022/1133882 | |
9. | Rita Shakouri, Maziar Salahi, Performance measurement and resource sharing among business sub-units in the presence of non-discretionary factors, 2022, 17, 1746-5664, 100, 10.1108/JM2-09-2020-0249 | |
10. | Shuai Wang, Cunyi Yang, Zhenghui Li, Spatio-Temporal Evolution Characteristics and Spatial Interaction Spillover Effects of New-Urbanization and Green Land Utilization Efficiency, 2021, 10, 2073-445X, 1105, 10.3390/land10101105 | |
11. | Pejman Peykani, Ali Emrouznejad, Emran Mohammadi, Jafar Gheidar-Kheljani, A novel robust network data envelopment analysis approach for performance assessment of mutual funds under uncertainty, 2022, 0254-5330, 10.1007/s10479-022-04625-3 | |
12. | Ulaş Ünlü, Neşe Yalçın, Nuri Avşarlıgil, Analysis of Efficiency and Productivity of Commercial Banks in Turkey Pre- and during COVID-19 with an Integrated MCDM Approach, 2022, 10, 2227-7390, 2300, 10.3390/math10132300 | |
13. | Alireza Amirteimoori, Leila Khoshandam, Sohrab Kordrostami, Monireh Jahani Sayyad Noveiri, Reza Kazemi Matin, Performance analysis in a stochastic supply chain with reverse flows: a DEA-based approach, 2022, 33, 1471-678X, 433, 10.1093/imaman/dpab018 | |
14. | M. Salahi, A. Jamalian, R. Shakouri, K. Asanimoghadam, Bikash Koli Dey, Additive Slack-Based Measure for a Two-Stage Structure with Shared Inputs and Undesirable Feedback, 2022, 2022, 1687-9155, 1, 10.1155/2022/7596736 | |
15. | Yi Sima, Jizheng Yi, Aibin Chen, Ze Jin, Automatic expression recognition of face image sequence based on key-frame generation and differential emotion feature, 2021, 113, 15684946, 108029, 10.1016/j.asoc.2021.108029 | |
16. | Saeed Yousefi, Hadi Shabanpour, Kian Ghods, Reza Farzipoor Saen, How to improve the future efficiency of Covid-19 treatment centers? A hybrid framework combining artificial neural network and congestion approach of data envelopment analysis, 2023, 176, 03608352, 108933, 10.1016/j.cie.2022.108933 | |
17. | Eleni-Maria Vretta, Kyriakos Bitsis, Konstantinos Kaparis, Georgios Paltayian, Andreas C. Georgiou, 2023, A Stochastic Bilevel DEA-Based Model for Resource Allocation, 40, 10.3390/IOCMA2023-14594 | |
18. | Andreas C. Georgiou, Konstantinos Kaparis, Eleni-Maria Vretta, Kyriakos Bitsis, George Paltayian, A Bilevel DEA Model for Efficiency Evaluation and Target Setting with Stochastic Conditions, 2024, 12, 2227-7390, 529, 10.3390/math12040529 | |
19. | Fatemeh Sadat Seyed Esmaeili, Emran Mohammadi, Amin Mostafaee, Z-number network data envelopment analysis approach: A case study on the Iranian insurance industry, 2024, 19, 1932-6203, e0306876, 10.1371/journal.pone.0306876 | |
20. | Rita Shakouri, Maziar Salahi, Yi-Kuei Lin, Malmquist–Luenberger Productivity Index for a Two-Stage Structure in the Presence of Undesirable Outputs and Uncertainty, 2024, 2024, 1687-9155, 1, 10.1155/2024/6905897 |
Ring type | Ring number | Strut number(each ring) | Strut height(mm) | Strut radius(mm) |
Type Ⅰ | 3 | 7 | 15 | 1.2 |
Type Ⅱ | 4 | 4, 5 | 14, 9 | 1.2 |
Type Ⅲ | 1 | 9 | 12 | 1.2 |
Specimen number | Factors | Performances | |||||||
A | B | C | D | E | Global flexibility | Local flexibility | Durability | ||
1 | 1 | 1 | 1 | 1 | 1 | RM | SV | LS | CS |
2 | 1 | 2 | 2 | 2 | 2 | RM | SV | LS | CS |
3 | 1 | 3 | 3 | 3 | 3 | RM | SV | LS | CS |
4 | 1 | 4 | 4 | 4 | 4 | RM | SV | LS | CS |
5 | 2 | 1 | 2 | 3 | 4 | RM | SV | LS | CS |
6 | 2 | 2 | 1 | 4 | 3 | RM | SV | LS | CS |
7 | 2 | 3 | 4 | 1 | 2 | RM | SV | LS | CS |
8 | 2 | 4 | 3 | 2 | 1 | RM | SV | LS | CS |
9 | 3 | 1 | 3 | 4 | 2 | RM | SV | LS | CS |
10 | 3 | 2 | 4 | 3 | 1 | RM | SV | LS | CS |
11 | 3 | 3 | 1 | 2 | 4 | RM | SV | LS | CS |
12 | 3 | 4 | 2 | 1 | 3 | RM | SV | LS | CS |
13 | 4 | 1 | 4 | 2 | 3 | RM | SV | LS | CS |
14 | 4 | 2 | 3 | 1 | 4 | RM | SV | LS | CS |
15 | 4 | 3 | 2 | 4 | 1 | RM | SV | LS | CS |
16 | 4 | 4 | 1 | 3 | 2 | RM | SV | LS | CS |
K1 | KA1 | KB1 | KC1 | KD1 | KE1 | ||||
K2 | KA2 | KB2 | KC2 | KD2 | KE2 | ||||
K3 | KA3 | KB3 | KC3 | KD3 | KE3 | ||||
K4 | KA4 | KB4 | KC4 | KD4 | KE4 | ||||
R | KmaxAi−KminAi | KmaxBi−KminBi | KmaxCi−KminCi | KmaxDi−KminDi | KmaxEi−KminEi |
![]() |
A | B | C | D | E |
1 | 0 | 0 | 1.2 mm | 0.55 mm | 0.1 mm |
2 | 2 | 2 | 1.5 mm | 0.65 mm | 0.12 mm |
3 | −2 | 4 | 0.8 mm | 0.45 mm | 0.08 mm |
4 | −4 | −2 | 0.6 mm | 0.35 mm | 0.06 mm |
Nitinol | |
Austenite Young's modulus EA (MPa) | 650000 |
Austenite Poisson's ratio μA | 0.33 |
Martensite Young's modulus EA (MPa) | 40000 |
Martensite Poisson's ratio μM | 0.33 |
Transformation strain εL | 0.041 |
Loading (δσ/δT)L(MPaT−1) | 6.7 |
Start of transformation loading σSL(MPa) | 440 |
End of transformation loading σEL(MPa) | 540 |
Reference temperature T0 (℃) | 22 |
Unloading (δσ/δT)U(MPaT−1) | 6.7 |
Start of transformation loading σSU(MPa) | 250 |
End of transformation loading σEU(MPa) | 140 |
Strain limit εmax | 12% |
e-PTFE graft | |
Young's modulus E (MPa) | 55.2 |
Poisson's ratio μ | 0.46 |
Ultimate strain εR | 20% |
Ring type | Ring number | Strut number(each ring) | Strut height(mm) | Strut radius(mm) |
Type Ⅰ | 3 | 7 | 15 | 1.2 |
Type Ⅱ | 4 | 4, 5 | 14, 9 | 1.2 |
Type Ⅲ | 1 | 9 | 12 | 1.2 |
Specimen number | Factors | Performances | |||||||
A | B | C | D | E | Global flexibility | Local flexibility | Durability | ||
1 | 1 | 1 | 1 | 1 | 1 | RM | SV | LS | CS |
2 | 1 | 2 | 2 | 2 | 2 | RM | SV | LS | CS |
3 | 1 | 3 | 3 | 3 | 3 | RM | SV | LS | CS |
4 | 1 | 4 | 4 | 4 | 4 | RM | SV | LS | CS |
5 | 2 | 1 | 2 | 3 | 4 | RM | SV | LS | CS |
6 | 2 | 2 | 1 | 4 | 3 | RM | SV | LS | CS |
7 | 2 | 3 | 4 | 1 | 2 | RM | SV | LS | CS |
8 | 2 | 4 | 3 | 2 | 1 | RM | SV | LS | CS |
9 | 3 | 1 | 3 | 4 | 2 | RM | SV | LS | CS |
10 | 3 | 2 | 4 | 3 | 1 | RM | SV | LS | CS |
11 | 3 | 3 | 1 | 2 | 4 | RM | SV | LS | CS |
12 | 3 | 4 | 2 | 1 | 3 | RM | SV | LS | CS |
13 | 4 | 1 | 4 | 2 | 3 | RM | SV | LS | CS |
14 | 4 | 2 | 3 | 1 | 4 | RM | SV | LS | CS |
15 | 4 | 3 | 2 | 4 | 1 | RM | SV | LS | CS |
16 | 4 | 4 | 1 | 3 | 2 | RM | SV | LS | CS |
K1 | KA1 | KB1 | KC1 | KD1 | KE1 | ||||
K2 | KA2 | KB2 | KC2 | KD2 | KE2 | ||||
K3 | KA3 | KB3 | KC3 | KD3 | KE3 | ||||
K4 | KA4 | KB4 | KC4 | KD4 | KE4 | ||||
R | KmaxAi−KminAi | KmaxBi−KminBi | KmaxCi−KminCi | KmaxDi−KminDi | KmaxEi−KminEi |
![]() |
A | B | C | D | E |
1 | 0 | 0 | 1.2 mm | 0.55 mm | 0.1 mm |
2 | 2 | 2 | 1.5 mm | 0.65 mm | 0.12 mm |
3 | −2 | 4 | 0.8 mm | 0.45 mm | 0.08 mm |
4 | −4 | −2 | 0.6 mm | 0.35 mm | 0.06 mm |
Nitinol | |
Austenite Young's modulus EA (MPa) | 650000 |
Austenite Poisson's ratio μA | 0.33 |
Martensite Young's modulus EA (MPa) | 40000 |
Martensite Poisson's ratio μM | 0.33 |
Transformation strain εL | 0.041 |
Loading (δσ/δT)L(MPaT−1) | 6.7 |
Start of transformation loading σSL(MPa) | 440 |
End of transformation loading σEL(MPa) | 540 |
Reference temperature T0 (℃) | 22 |
Unloading (δσ/δT)U(MPaT−1) | 6.7 |
Start of transformation loading σSU(MPa) | 250 |
End of transformation loading σEU(MPa) | 140 |
Strain limit εmax | 12% |
e-PTFE graft | |
Young's modulus E (MPa) | 55.2 |
Poisson's ratio μ | 0.46 |
Ultimate strain εR | 20% |