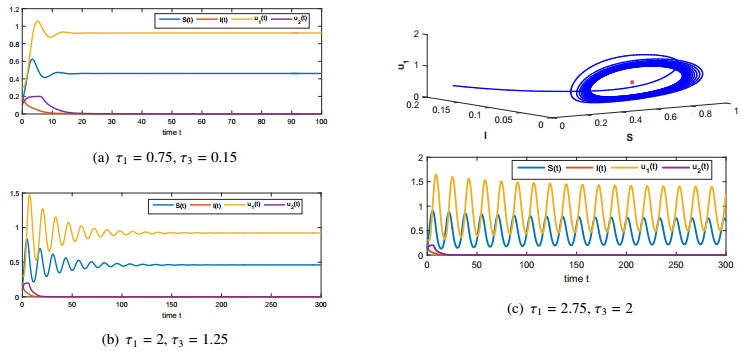
Citation: Stefan G. Hofmann, Elizabeth A. Mundy, Joshua Curtiss. Neuroenhancement of Exposure Therapy in Anxiety Disorders[J]. AIMS Neuroscience, 2015, 2(3): 123-138. doi: 10.3934/Neuroscience.2015.3.123
[1] | Cruz Vargas-De-León, Alberto d'Onofrio . Global stability of infectious disease models with contact rate as a function of prevalence index. Mathematical Biosciences and Engineering, 2017, 14(4): 1019-1033. doi: 10.3934/mbe.2017053 |
[2] | Yoichi Enatsu, Yukihiko Nakata, Yoshiaki Muroya . Global stability for a class of discrete SIR epidemic models. Mathematical Biosciences and Engineering, 2010, 7(2): 347-361. doi: 10.3934/mbe.2010.7.347 |
[3] | Jinliang Wang, Hongying Shu . Global analysis on a class of multi-group SEIR model with latency and relapse. Mathematical Biosciences and Engineering, 2016, 13(1): 209-225. doi: 10.3934/mbe.2016.13.209 |
[4] | Rongjian Lv, Hua Li, Qiubai Sun, Bowen Li . Model of strategy control for delayed panic spread in emergencies. Mathematical Biosciences and Engineering, 2024, 21(1): 75-95. doi: 10.3934/mbe.2024004 |
[5] | Yu Ji . Global stability of a multiple delayed viral infection model with general incidence rate and an application to HIV infection. Mathematical Biosciences and Engineering, 2015, 12(3): 525-536. doi: 10.3934/mbe.2015.12.525 |
[6] | Abdennasser Chekroun, Mohammed Nor Frioui, Toshikazu Kuniya, Tarik Mohammed Touaoula . Global stability of an age-structured epidemic model with general Lyapunov functional. Mathematical Biosciences and Engineering, 2019, 16(3): 1525-1553. doi: 10.3934/mbe.2019073 |
[7] | Gonzalo Robledo . Feedback stabilization for a chemostat with delayed output. Mathematical Biosciences and Engineering, 2009, 6(3): 629-647. doi: 10.3934/mbe.2009.6.629 |
[8] | Pan Yang, Jianwen Feng, Xinchu Fu . Cluster collective behaviors via feedback pinning control induced by epidemic spread in a patchy population with dispersal. Mathematical Biosciences and Engineering, 2020, 17(5): 4718-4746. doi: 10.3934/mbe.2020259 |
[9] | Dongmei Li, Bing Chai, Weihua Liu, Panpan Wen, Ruixue Zhang . Qualitative analysis of a class of SISM epidemic model influenced by media publicity. Mathematical Biosciences and Engineering, 2020, 17(5): 5727-5751. doi: 10.3934/mbe.2020308 |
[10] | Jianquan Li, Zhien Ma, Fred Brauer . Global analysis of discrete-time SI and SIS epidemic models. Mathematical Biosciences and Engineering, 2007, 4(4): 699-710. doi: 10.3934/mbe.2007.4.699 |
Infectious diseases (such as influenza, malaria, cholera, tuberculosis, hepatitis, AIDS, etc) have always seriously threatened humans' life and health. With in-depth understanding of infectious diseases, scientists have been continuing to explore effective methods to prevent and control the outbreaks of various infectious diseases. It is well known that mathematical models have played very important roles in analysis of control strategies for disease transmission [1,2,3,4,5,6,7,8,9,10].
When studying the long-term evolutionary behavior of an ecological system, as pointed in [11], the equilibrium of biological system may not be the desirable one, and smaller value is required. This can be achieved by introducing suitable feedback control variable. The feedback control mechanism might be implemented through harvesting or culling procedures or certain biological control schemes [12]. In addition, in a control system, the time delay factor generally exists in the signal transmission process. Thus, feedback control with coupled time delay may have better biological significance [12] and has been extensively introduced into some important population ecological systems (see, for example, [13,14,15,16] and the references cited therein).
In recent years, feedback control has also been successfully applied to some infectious disease dynamical systems. For example, in [17], the authors considered the following SI epidemic model with two feedback control variables:
$ {˙S(t)=S(t)(r−aS(t)−bI(t)−c1u1(t)),˙I(t)=I(t)(bS(t)−μ−fI(t)−c2u2(t)),˙u1(t)=−e1u1(t)+d1S(t),˙u2(t)=−e2u2(t)+d2I(t). $
|
(1.1) |
In model (1.1), the state variables $ S(t) $ and $ I(t) $ represent the numbers of susceptibles and infectives at time $ t $, respectively; $ u_{1}(t) $ and $ u_{2}(t) $ are feedback control variables. The number of susceptibles grows according to the regulation of a logistic curve with the capacity $ r/a $ ($ r > 0 $, $ a > 0 $) and a constant recruitment rate $ r $; the constant $ b > 0 $ is the transmission rate when susceptibles contact with infectives; the constants $ \mu > 0 $ and $ f > 0 $ are the death rates of the infectives with respect to single and mutiple of infectives, respectively; the constants $ c_1 > 0 $, $ c_2 > 0 $, $ d_1 > 0 $, $ d_2 > 0 $, $ e_1 > 0 $ and $ e_2 > 0 $ are the feedback control parameters. By constructing suitable Lyapunov functions, the authors established threshold dynamics of model (1.1) completely determined by the threshold parameter $ \gamma_{0} = (br-a\mu)e_1/(c_1d_1\mu) $. The results in [17] indicate that, by appropriately choosing feedback control parameters, it can make the disease infection endemic or extinct. In [18], the author considered a two-group SI epidemic model with feedback control only in the susceptible individuals, and showed that the disease outbreaks can be controlled by adjusting feedback control parameters. In addition, in [19], the authors further extend model (1.1) to the case of patchy environment.
Since the authors of [20] have introduced a nonlinear incidence rate $ g(I)S $ into classic Kermack-McKendrick SIR model, nonlinear incidence rate has been further introduced into more general SIR/SIRS epidemic models with time delays or infection age etc (see, for example, [21,22,23,24,25,26,27] and the references cited therein). Usually, the function $ g(I) $ takes the following two types: (ⅰ) saturated, such as $ g(I) = bI/(1+kI) $, or $ g(I) = bI^2/(1+kI^2) $; (ⅱ) unimodal, such as $ g(I) = bI/(1+kI^2) $, here $ k > 0 $ is constant. In biology, $ bI $ or $ bI^2 $ measures the infection force of the disease, $ 1/(1+kI) $ or $ 1/(1+kI^2) $ measures the inhibition effect from the behavioral change of the susceptible individuals when their number increases or from the crowding effect of the infective individuals [21,22].
Recently, in [28], the authors further extended model (1.1) to the following more general case with the saturated incidence rate $ bSI/(1+kI) $ and feedback controls:
$ {˙S(t)=S(t)(r−aS(t)−bI(t)1+kI(t)−c1u1(t)),˙I(t)=I(t)(bS(t)1+kI(t)−μ−fI(t)−c2u2(t)),˙u1(t)=−e1u1(t)+d1S(t),˙u2(t)=−e2u2(t)+d2I(t), $
|
(1.2) |
and some sufficient conditions for global asymptotic stability of the disease-free equilibrium and the endemic equilibrium of model (1.2) are established by the method of Lyapunov functions. In addition, the authors also considered permanence and existence of almost periodic solutions for a class of non-autonomous system based on model (1.2).
Motivated by the above works and model (1.2), we further consider the following SI epidemic model with saturated incidence rate, two feedback control variables and four time delays:
$ {˙S(t)=S(t)(r−aS(t)−bI(t)1+kI(t)−c1u1(t−τ1)),˙I(t)=I(t)(bS(t)1+kI(t)−μ−fI(t)−c2u2(t−τ2)),˙u1(t)=−e1u1(t)+d1S(t−τ3),˙u2(t)=−e2u2(t)+d2I(t−τ4). $
|
(1.3) |
The biological significance of all the parameters of model (1.3) are the same as in model (1.2) except time delays $ \tau_{i}\geq0 $ ($ i = 1, 2, 3, 4 $). In model (1.3), $ u_1(t) $ and $ u_2(t) $ are introduced as control variables. Usually, there always exist time delays in the transmission of information. Therefore, $ \tau_1 $ and $ \tau_2 $ can be understood as the result of transmission of information, and while $ \tau_3 $ and $ \tau_4 $ represent usual feedback control delays.
The purpose in this paper focuses on global dynamics of the equilibria of model (1.3) by constructing appropriate Lyapunov functionals, and our results further extend and improve works in [17,28].
The rest of the paper is organized as follows. In Section 2, we provide some preliminary results, including the well-posedness and dissipativeness of the solutions of model (1.3), the expression of the basic reproduction number and the classification of the equilibria of model (1.3). In Sections 3 and 4, we establish some sufficient conditions for global asymptotic stability and global attractivity of the disease-free equilibrium and the endemic equilibrium of model (1.3), which are the main results of this paper. In the last section, the conclusions and some numerical simulations are given.
Let $ C^{+} = C([-\tau, 0], \mathbb{R}_{+}^{4}) $ be the Banach space of continuous functions mapping the interval $ [-\tau, 0] $ into $ \mathbb{R}_{+}^{4} $ equipped with the supremum norm, where $ \tau = \max\{\tau_1, \tau_2, \tau_3, \tau_4\} $. The initial condition of model (1.3) is given as follows,
$ S(θ)=ϕ1(θ),I(θ)=ϕ2(θ),u1(θ)=ϕ3(θ),u2(θ)=ϕ4(θ),θ∈[−τ,0], $
|
(2.1) |
where $ \phi = (\phi_{1}(\theta), \phi_{2}(\theta), \phi_{3}(\theta), \phi_{4}(\theta))\in C^{+} $.
By using the standard theory of delay differential equations (DDEs) (see, for example, [29,30,31]), we can easily establish the following result.
Theorem 2.1. The solution $ (S(t), I(t), u_{1}(t), u_{2}(t)) $ of model (1.3) with the initial condition (2.1) is existent, unique and nonnegative on $ [0, \infty) $, and satisfies
$ lim supt→+∞S(t)≤ra,lim supt→+∞I(t)≤rbaf,lim supt→+∞u1(t)≤rd1ae1,lim supt→+∞u2(t)≤rbd2afe2. $
|
(2.2) |
Moreover, the following bounded set
$ \Omega: = \left\{\phi\in C^{+}: \; \|\phi_{1}\|\leq\frac{r}{a},\; \|\phi_{2}\|\leq\frac{rb}{af},\; \|\phi_{3}\|\leq\frac{rd_{1}}{ae_{1}},\; \|\phi_{4}\|\leq \frac{rbd_{2}}{afe_{2}} \right\} $ |
is positively invariant with respect to model (1.3).
Proof. It is not difficult to show that the solution $ (S(t), I(t), u_{1}(t), u_{2}(t)) $ of model (1.3) with the initial condition (2.1) is existent, unique and nonnegative on $ [0, \infty) $. Let us consider ultimate boundedness of model (1.3). According to the first equation of model (1.3), we have that for $ t\geq 0 $,
$ ˙S(t)≤S(t)(r−aS(t)), $
|
(2.3) |
which implies $ \limsup_{t\rightarrow+\infty}S(t)\leq \frac{r}{a} $. For any sufficiently small $ \varepsilon > 0 $, there exists a $ \widehat{t} > 0 $ such that $ S(t) < \frac{r}{a}+\varepsilon $ for $ t\geq \widehat{t} $. Further, according to the second equation of model (1.3), we have for $ t\geq \widehat{t} $,
$ { \dot{I}(t)\leq I(t)\left[b\left(\frac{r}{a}+\varepsilon\right)-fI(t)\right], } $ |
which implies
$ lim supt→+∞I(t)≤rbaf+bfε. $
|
(2.4) |
Since inequality (2.4) holds for arbitrary $ \varepsilon > 0 $, we obtain $ \limsup_{t\rightarrow+\infty}I(t)\leq \frac{rb}{af} $. Similarly, according to the last two equations of model (1.3), we can obtain $ \limsup_{t\rightarrow+\infty}u_{1}(t)\leq\frac{rd_{1}}{ae_{1}} $, $ \limsup_{t\rightarrow+\infty}u_{2}(t)\leq\frac{rbd_{2}}{afe_{2}} $.
Let $ (S(t), I(t), u_{1}(t), u_{2}(t)) $ be the solution of model (1.3) with the initial function $ \phi = (\phi_{1}, \phi_{2}, \phi_{3}, \phi_{4})\in \Omega $. { For $ t\geq0 $, we have $ \dot{S}(t)\leq S(t)\left(r-aS(t)\right) $, which implies that for $ t\geq0 $,
$ S(t)≤raϕ1(0)ϕ1(0)+[ra−ϕ1(0)]e−rt≤ra, $
|
where $ \phi_{1}(0)\leq \frac{r}{a} $ is used. Further combining the second equation of model (1.3), for $ t\geq0 $, we have $ \dot{I}(t)\leq I(t)(\frac{rb}{a}-fI(t)) $, which implies that for $ t\geq0 $,
$ I(t)≤rbafϕ2(0)ϕ2(0)+[rbaf−ϕ2(0)]e−rbat≤rbaf, $
|
where $ \phi_{2}(0)\leq \frac{rb}{af} $ is used. Thus, for $ t\geq0 $, we have $ \dot{u}_{1}(t)\leq \frac{rd_{1}}{a}-e_{1}u_{1}(t), \; \; \dot{u}_{2}(t) \leq \frac{rbd_{2}}{af}-e_{2}u_{2}(t) $. This implies that for $ t\geq0 $,
$ u1(t)≤rd1ae1+[ϕ3(0)−rd1ae1]e−e1t≤rd1ae1,u2(t)≤rbd2afe2+[ϕ4(0)−rbd2afe2]e−e2t≤rbd2afe2, $
|
where $ \phi_{3}(0)\leq \frac{rd_{1}}{ae_{1}} $ and $ \phi_{4}(0)\leq \frac{rbd_{2}}{afe_{2}} $ are used. Hence, it has that $ \Omega $ is positively invariant with respect to model (1.3).
The proof is completed.
Obviously, model (1.3) always has a trivial equilibrium $ \widetilde{E} = (0, 0, 0, 0) $ and a disease-free equilibrium $ E_{0} = (S^{0}, 0, u_{1}^{0}, 0) $, where
$ S^{0} = \frac{re_{1}}{ae_{1}+d_{1}c_{1}}, \; \; u_{1}^{0} = \frac{rd_{1}}{ae_{1}+d_{1}c_{1}}. $ |
Then, by the methods in [32,33], we can derive the expression of the basic reproduction number of model (1.3) as follows. First we define matrices $ \mathbb{F} $ and $ \mathbb{V} $ as
$ \mathbb{F} = \left( bS0000 \right),\; \; \; \mathbb{V} = \left( μ0−d2e2 \right). $
|
Then the basic reproduction number $ R_0 $ is defined as the spectral radius of $ \mathbb{F}\mathbb{V}^{-1} $. Therefore,
$ R_{0}: = \rho(\mathbb{F}\mathbb{V}^{-1}) = \frac{bS^{0}}{\mu} = \frac{bre_{1}}{\mu(ae_{1}+d_{1}c_{1})}. $ |
Suppose $ (S, I, u_{1}, u_{2}) $ is an endemic equilibrium (positive equilibrium) of model (1.3), where $ S > 0 $, $ I > 0 $, $ u_{1} > 0 $, $ u_{2} > 0 $ satisfy the following equations
$ r−aS−bI1+kI−c1u1=0,bS1+kI−μ−fI−c2u2=0,−e1u1+d1S=0,−e2u2+d2I=0. $
|
(2.5) |
From Eq (2.5), it is not difficult to obtain the following relationships
$ u2=d2e2I,u1=d1e1S,S=e1ae1+d1c1(r−bI1+kI). $
|
(2.6) |
Through Eq (2.6) and combining the second equation of Eq (2.5), we can obtain that $ I $ satisfies the following equation,
$ F(I)\equiv \frac{be_{1}}{(ae_{1}+d_{1}c_{1})(1+kI)} \left(r-\frac{bI}{1+kI}\right)-\mu-\left(f+\frac{d_{2}c_{2}}{e_{2}}\right)I = 0. $ |
According to Eq (2.6), in order to ensure that $ S > 0 $, we need to consider the following two cases:
(ⅰ) $ rk < b $, $ 0 < I < \frac{r}{b-rk}\equiv \widetilde{I} $;
(ⅱ) $ rk\geq b $, $ I > 0 $.
Clearly, for both case (ⅰ) and case (ⅱ), we have that
$ \dot{F}(I) = -\frac{be_{1}k}{(ae_{1}+d_{1}c_{1})(1+kI)^{2}} \left(r-\frac{bI}{1+kI}\right) -\frac{b^{2}e_{1}}{(ae_{1}+d_{1}c_{1})(1+kI)^{3}} -\left(f+\frac{d_{2}c_{2}}{e_{2}}\right) \lt 0. $ |
Hence, $ F(I) $ is monotonically decreasing with respect to $ I $ and
$ \lim\limits_{I\rightarrow 0^{+}}F(I) = \frac{bre_{1}}{ae_{1}+d_{1}c_{1}}-\mu = \mu(R_{0}-1). $ |
If $ R_{0}\leq1 $, then $ \lim_{I\rightarrow 0^{+}}F(I)\leq0 $ and $ F(I) = 0 $ has no positive roots. If $ R_{0} > 1 $, then $ \lim_{I\rightarrow 0^{+}}F(I) > 0 $.
For case (ⅰ), we have that
$ \lim\limits_{I\rightarrow \widetilde{I}^{-}}F(I) = -\mu-\left(f+\frac{d_{2}c_{2}}{e_{2}}\right)\widetilde{I} \lt 0. $ |
For case (ⅱ), we have that
$ F\left(\frac{rbe_{1}e_{2}}{(ae_{1}+d_{1}c_{1})(fe_{2}+d_{2}c_{2})}\right) \lt \frac{rbe_{1}}{ae_{1}+d_{1}c_{1}} -\left(f+\frac{d_{2}c_{2}}{e_{2}}\right) \frac{rbe_{1}e_{2}}{(ae_{1}+d_{1}c_{1})(fe_{2}+d_{2}c_{2})} = 0. $ |
Therefore, for cases (ⅰ) and (ⅱ), $ F(I) = 0 $ has a unique positive root $ I = I^{*} $ if $ R_{0} > 1 $.
From the above discussions, we have the following result.
Theorem 2.2. The following statements are true.
(i) Model (1.3) always has a trivial equilibrium $ \widetilde{E} = (0, 0, 0, 0) $.
(ii) Model (1.3) always has a disease-free equilibrium $ E_{0} = (S^{0}, 0, u_{1}^{0}, 0) $.
(iii) Only for $ R_{0} > 1 $, model (1.3) has a unique endemic equilibrium $ E^{*} = (S^{*}, I^{*}, u_{1}^{*}, u_{2}^{*}) $, where
$ S^{*} = \frac{e_{1}}{ae_{1}+d_{1}c_{1}}\left(r-\frac{bI^{*}}{1+kI^{*}}\right), \; \; u_{1} = \frac{d_{1}}{e_{1}}S^{*}, \; \; u_{2} = \frac{d_{2}}{e_{2}}I^{*}, $ |
and $ I^{*} $ is the unique positive root of the equation $ F(I) = 0 $.
Remak 2.1. In fact, the classifications of the equilibria of models (1.2) and (1.3) are exactly the same for any $ \tau_{i}\geq0 $. Clearly, comparing with the reference [28], our Theorem 2.2 gives more complete classification of the equilibria of model (1.2) and clearer expression of the basic reproduction number $ R_0 $.
Let $ \overline{E} = (\overline{S}, \overline{I}, \overline{u}_{1}, \overline{u}_{2}) $ be any equilibrium of model (1.3). In order to investigate local stability of the equilibrium $ \overline{E} $, we easily have that the characteristic equation of the corresponding linearized system of model (1.3) at $ \overline{E} $ is given by
$ |λ−(r−2a¯S−b¯I1+k¯I−c1¯u1)b¯S(1+k¯I)2c1¯Se−λτ10−b¯I1+k¯Iλ−(b¯S(1+k¯I)2−μ−2f¯I−c2¯u2)0c2¯Ie−λτ2−d1e−λτ30λ+e100−d2e−λτ40λ+e2|=0. $
|
(3.1) |
At the trivial equilibrium $ \widetilde{E} = (0, 0, 0, 0) $, the characteristic Eq (3.1) becomes
$ (\lambda-r)(\lambda+\mu)(\lambda+e_{1})(\lambda+e_{2}) = 0, $ |
which has a positive real root $ \lambda = r $. Hence, $ \widetilde{E} $ is unstable for any $ \tau_{i}\geq0 $ ($ i = 1, 2, 3, 4 $).
At the disease-free equilibrium $ E_{0} = (S^{0}, 0, u^{0}_{1}, 0) $, the characteristic Eq (3.1) becomes
$ (λ+μ−bS0)(λ+e2)[λ2+(aS0+e1)λ+ae1S0+d1c1S0e−λ(τ1+τ3)]=0. $
|
(3.2) |
It is clear that Eq (3.2) has two real roots $ \lambda_{1} = -e_{2} < 0 $ and $ \lambda_{2} = -\mu+bS^{0} = \mu(R_{0}-1) $. Obviously, when $ R_0 > 1 $, Eq (3.2) has a positive real root $ \lambda_{2} > 0 $, and hence, $ E_{0} $ is unstable for any $ \tau_{i}\geq0 $ ($ i = 1, 2, 3, 4 $). When $ R_0 < 1 $, then $ \lambda_{2} < 0 $. When $ R_0 = 1 $, then $ \lambda_{2} = 0 $ is a simple root of Eq (3.2).
Let
$ F1(λ,τ1,τ3)≡λ2+(aS0+e1)λ+ae1S0+d1c1S0e−λ(τ1+τ3)=0. $
|
(3.3) |
The distribution of the roots of Eq (3.3) in the complex plane has been discussed in detail in [30,31,34]. Therefore, we have the following conclusions.
Lemma 3.1. The following statements are true.
(i) If $ d_{1}c_{1}\leq ae_{1} $, then all the roots of Eq (3.3) have negative real parts.
(ii) If $ d_{1}c_{1} > ae_{1} $, then all the roots of Eq (3.3) have negative real parts for $ \tau_{1}+\tau_{3} < \tau_{13}^{0} $, and Eq (3.3) has at least one root which has positive real part for $ \tau_{1}+\tau_{3} > \tau_{13}^{0} $, where
$ \tau_{13}^{0} = \frac{1}{\omega}\arccos\left[ \frac{\omega^{2}-ae_{1}S^{0}}{d_{1}c_{1}S^{0}}\right], $ |
$ \omega = \left(\frac{-\left[(aS^{0})^{2}+e_{1}^{2}\right] +\sqrt{\left[(aS^{0})^{2}+e_{1}^{2}\right]^{2}-4(S^{0})^{2}(ae_{1}+d_{1}c_{1}) (ae_{1}-d_{1}c_{1})}}{2}\right)^{\frac{1}{2}}. $ |
According to the discussions above and Lemma 3.1, it follows from stability theory and Hopf bifurcation theorem for DDEs (see, for example, [29,30,31]) that the following results hold.
Theorem 3.1. The trivial equilibrium $ \widetilde{E} $ of model (1.3) is unstable for any $ \tau_{i}\geq0 \; (i=1,2,3,4
Theorem 3.2. For any $ \tau_{2}\geq0 $ and $ \tau_{4}\geq0 $, the following statements are true.
(i) If $ R_{0} > 1 $, then the disease-free equilibrium $ E_{0} $ is unstable for any $ \tau_{1}\geq0 $ and $ \tau_{3}\geq0 $.
(ii) Assume that $ d_{1}c_{1}\leq ae_{1} $. If $ R_{0} < 1 $, then the disease-free equilibrium $ E_{0} $ is locally asymptotically stable for any $ \tau_{1}\geq0 $ and $ \tau_{3}\geq0 $; If $ R_{0} = 1 $, then the disease-free equilibrium $ E_{0} $ is linearly stable for any $ \tau_{1}\geq0 $ and $ \tau_{3}\geq0 $.
(iii) Assume that $ d_{1}c_{1} > ae_{1} $. If $ R_{0} < 1 $, then the disease-free equilibrium $ E_{0} $ is locally asymptotically stable for $ \tau_{1}+\tau_{3} < \tau_{13}^{0} $, and is unstable for $ \tau_{1}+\tau_{3} > \tau_{13}^{0} $. Moreover, model (1.3) undergoes a Hopf bifurcation at the disease-free equilibrium $ E_{0} $ when $ \tau_{1}+\tau_{3} = \tau_{13}^{0} $.
Remak 3.1. Theorem 3.2 indicates that time delays $ \tau_2 $ and $ \tau_4 $ do not affect local asymptotic stability of the disease-free equilibrium $ E_{0} $, and under the condition of $ d_{1}c_{1}\leq ae_{1} $, time delays $ \tau_1 $ and $ \tau_3 $ also do not affect local asymptotic stability of $ E_{0} $. But under the condition $ d_{1}c_{1} > ae_{1} $, for larger time delay $ \tau_1 $ or $ \tau_3 $, stability of $ E_0 $ will be lost.
In the following discussions, we establish some sufficient conditions for global asymptotic stability of the disease-free equilibrium $ E_{0} $.
Theorem 3.3. Assume that $ d_{1}c_{1}\leq ae_{1} $. For any $ \tau_{i}\geq0 $ $ (i = 1, 2, 3, 4) $, the following statements are true.
(i) If $ R_{0} < 1 $, then the disease-free equilibrium $ E_{0} $ is globally asymptotically stable in $ \Omega_{1}: = \{\phi\in \Omega:\phi_{1}(0) > 0\} $.
(ii) If $ R_{0} = 1 $, then the disease-free equilibrium $ E_{0} $ is globally attractive in $ \Omega_{1} $.
Proof. First, it is easy to show that the set $ \Omega_{1} $ is positively invariant for model (1.3). If $ R_0 < 1 $, by Theorem 3.2, we only need to show that the disease-free equilibrium $ E_{0} $ is globally attractive.
Define a Lyapunov functional $ L_{1} $ on $ \Omega_{1} $ as follows,
$ L_{1} = V_{1} +\frac{a}{2}\int_{-\tau_{3}}^{0}(\phi_1(\xi)-S^{0})^{2}d\xi +\frac{c_1e_{1}}{2d_1}\int_{-\tau_{1}}^{0} (\phi_{3}(\xi)-u_{1}^{0})^{2}d\xi, $ |
where
$ V_{1} = \phi_1(0)-S^{0}-S^{0}\ln\frac{\phi_1(0)}{S^{0}}+\phi_2(0) +\frac{c_1}{2d_1}(\phi_{3}(0)-u_{1}^{0})^{2}. $ |
It is clear that $ L_{1} $ is continuous on $ \Omega_{1} $ and satisfies the condition (ii) of Lemma 3.1 in [35] on $ \partial \Omega_{1} = \overline{\Omega}_{1}\setminus \Omega_{1} $.
Calculating the derivative of $ L_{1} $ along any solution $ (S(t), I(t), u_{1}(t), u_{2}(t)) $ of model (1.3), it follows that, for $ t\geq0 $,
$ dL1dt=dV1dt+a2(S(t)−S0)2−a2(S(t−τ3)−S0)2+c1e12d1(u1(t)−u01)2−c1e12d1(u1(t−τ1)−u01)2, $
|
(3.4) |
where
$ dV1dt=(S(t)−S0)[a(S0−S(t))−bI(t)1+kI(t)+c1(u01−u1(t−τ1))]+I(t)[bS(t)1+kI(t)−μ−fI(t)−c2u2(t−τ2)]+c1d1(u1(t)−u01)[−e1(u1(t)−u01)+d1(S(t−τ3)−S0)]=−a(S(t)−S0)2−[μ−bS01+kI(t)]I(t)−fI2(t)−c2I(t)u2(t−τ2)−c1e1d1(u1(t)−u01)2+c1(S(t)−S0)(u01−u1(t−τ1))+c1(u1(t)−u01)(S(t−τ3)−S0), $
|
(3.5) |
here $ r = aS^{0}+c_{1}u_{1}^{0} $ and $ e_{1}u_{1}^{0} = d_{1}S^{0} $ are used. Using the following inequality of arithmetic and geometric means,
$ Λ1≡c1(S(t)−S0)(u01−u1(t−τ1))+c1(u1(t)−u01)(S(t−τ3)−S0)≤√d1c1ae1[a2(S(t)−S0)2+c1e12d1(u1(t−τ1)−u01)2+a2(S(t−τ3)−S0)2+c1e12d1(u1(t)−u01)2], $
|
we further have that
$ dL1dt≤−a2(1−√d1c1ae1)[(S(t)−S0)2+(S(t−τ3)−S0)2]−[μ−bS01+kI(t)]I(t)−fI2(t)−c2I(t)u2(t−τ2)−c1e12d1(1−√d1c1ae1)[(u1(t)−u01)2+(u1(t−τ1)−u01)2]. $
|
(3.6) |
Note that, if $ R_{0}\leq1 $, we have that
$ −[μ−bS01+kI(t)]I(t)=−[μ−bS0+μkI(t)1+kI(t)]I(t)=−[μ(1−R0)1+kI(t)+μkI(t)1+kI(t)]I(t)≤0. $
|
(3.7) |
Assume that $ d_{1}c_{1} < ae_{1} $ and $ R_{0}\leq1 $. By inequalities (3.6) and (3.7), we can obtain that $ \frac{dL_{1}}{dt}\leq0 $ for $ t\geq0 $. Let $ M $ be the largest invariant set in the following set $ G $:
$ G: = \left\{\phi\in \overline{\Omega}_{1}:\; L_{1} \lt \infty \; \text{and}\; \frac{dL_{1}}{dt} = 0\right\}. $ |
Then, it follows from inequalities (3.6) and (3.7) that
$ G\subset \left\{\phi\in \Omega_{1}: \; \phi_{1}(0) = S^{0},\; \phi_{2}(0) = 0,\; \phi_{3}(0) = u_{1}^{0}\right\}. $ |
We can easily have from model (1.3) and the invariance of $ M $ that $ M = \{E_{0}\} $. Therefore, it follows from Lemma 3.1 in [35] that the disease-free equilibrium $ E_{0} $ is globally attractive.
Assume that $ d_{1}c_{1} = ae_{1} $ and $ R_{0}\leq1 $. In this case, it is not easy to conclude that the largest invariant set $ M $ is the singleton $ \{E_{0}\} $. Hence, it is necessary to analyze Eq (3.4).
Note that
$ −a2(S(t)−S0)2−c1e12d1(u1(t−τ1)−u01)2+c1(S(t)−S0)(u01−u1(t−τ1))=−d1c12e1(S(t)−S0+e1d1(u1(t−τ1)−u01))2,−a2(S(t−τ3)−S0)2−c1e12d1(u1(t)−u01)2+c1(u1(t)−u01)(S(t−τ3)−S0)=−d1c12e1(S(t−τ3)−S0−e1d1(u1(t)−u01))2=−d1c12e1(S(t−τ3)−e1d1u1(t))2. $
|
Hence, Eq (3.4) can be rewritten as
$ dL1dt=−d1c12e1[(S(t)−S0+e1d1(u1(t−τ1)−u01))2+(S(t−τ3)−e1d1u1(t))2]−[μ(1−R0)1+kI(t)+μkI(t)1+kI(t)]I(t)−fI2(t)−c2I(t)u2(t−τ2). $
|
(3.8) |
By inequality (3.7) and Eq (3.8), we can obtain that $ \frac{dL_{1}}{dt}\leq0 $ for $ t\geq0 $. Let $ M_{1} $ be the largest invariant set in the following set $ G_1 $:
$ G_{1}: = \left\{\phi\in \overline{\Omega}_{1}:\; L_{1} \lt \infty \; \text{and}\; \frac{dL_{1}}{dt} = 0\right\}. $ |
Then, it follows from Eq (3.8) that
$ G_{1}\subset \left\{\phi\in \Omega_{1}: \; \phi_{1}(0)+\frac{e_{1}}{d_{1}}\phi_{3}(-\tau_{1}) = S^{0}+\frac{e_{1}}{d_{1}}u_{1}^{0}, \; \phi_{1}(-\tau_{3})-\frac{e_{1}}{d_{1}}\phi_{3}(0) = 0, \; \phi_{2}(0) = 0\right\}. $ |
For any $ \varphi = (\varphi_{1}, \varphi_{2}, \varphi_{3}, \varphi_{4})\in M_{1} $, let $ (S(t), I(t), u_{1}(t), u_{2}(t)) $ be the solution of model (1.3) with the initial function $ \varphi $. From the invariance of $ M_{1} $, we have that $ (S_{t}, I_{t}, u_{1t}, u_{2t})\in M_{1}\subset G_{1} $ for any $ t\in R $. Obviously, $ I(t)\equiv0 $ for any $ t\in R $, and then from the fourth equation of model (1.3) and the invariance of $ M_{1} $, it is not difficult to obtain $ u_{2}(t)\equiv0 $ for any $ t\in R $. In addition, according to the first and third equations of model (1.3), we can obtain, for any $ t\in R $,
$ ˙S(t)=S(t)(r−aS(t)−c1u1(t−τ1))=S(t)(r−d1c1e1S(t)−c1u1(t−τ1))=0,˙u1(t)=−e1u1(t)+d1S(t−τ3)=0. $
|
Thus, there exist constants $ \delta_1 $ and $ \delta_2 $ such that $ S(t)\equiv \delta_{1} $ and $ u_{1}(t)\equiv \delta_{2} $ for any $ t\in R $. It is not difficult to find that $ \delta_1 $ and $ \delta_2 $ satisfy
$ \delta_{1}+\frac{e_{1}}{d_{1}}\delta_{2} = S^{0}+\frac{e_{1}}{d_{1}}u_{1}^{0}, \; \; \delta_{1}-\frac{e_{1}}{d_{1}}\delta_{2} = 0, $ |
which imply that $ \delta_1 = S^{0} $ and $ \delta_2 = u_{1}^{0} $. Hence, $ S(t)\equiv S^{0} $ and $ u_{1}(t)\equiv u_{1}^{0} $ for any $ t\in R $. This shows that $ M_{1} = \{E_{0}\} $. Then, it follows from Lemma 3.1 in [35] that the disease-free equilibrium $ E_{0} $ is globally attractive.
The proof is completed.
Remak 3.2. Note the conclusion (ii) of Theorem 3.2, where we see that Theorem 3.3 gives complete conclusion of the global dynamics of the disease-free equilibrium $ E_{0} $ in the case of $ d_{1}c_{1}\leq ae_{1} $.
Now, we continue to discuss global dynamics of the disease-free equilibrium $ E_{0} $ in the absence of condition $ d_{1}c_{1}\leq ae_{1} $. The following lemmas will be used.
Lemma 3.2. $ ($Barbalat's lemma [37,36]$) $ Let $ x(t) $ be a real valued differentiable function defined on some half line $ [a, +\infty) $, $ a\in(-\infty, +\infty) $. If
(i) $ \lim_{t\rightarrow+\infty}x(t) = \alpha $; $ |\alpha| < \infty $.
(ii) $ \dot{x}(t) $ is uniformly continuous for $ t > a $.
Then $ \lim_{t\rightarrow+\infty}\dot{x}(t) = 0 $.
Lemma 3.3. Let $ (S(t), I(t), u_{1}(t), u_{2}(t)) $ be any solution of model (1.3) with the initial condition (2.1), then the following statements are true.
(i) If $ rb\leq a\mu $ (which implies $ R_{0} < 1 $), then $ \lim_{t\rightarrow+\infty}I(t) = 0 $, $ \lim_{t\rightarrow+\infty}u_{2}(t) = 0 $.
(ii) If $ rb > a\mu $, then $ \limsup_{t\rightarrow+\infty}I(t)\leq I_{M} $, where
$ I_{M} = \left\{ √(kμ+f)2+4fk(bra−μ)−(kμ+f)2fk>0,k>0,rb−aμaf,k=0. \right. $
|
Proof. By inequality (2.2), for arbitrary $ \varepsilon > 0 $, there exists a $ T > 0 $ such that $ S(t) < \frac{r}{a}+\varepsilon $ for $ t > T $. Then, it follows from the second equation of model (1.3) that, for $ t > T $,
$ ˙I(t)≤I(t)(b(ra+ε)1+kI(t)−μ−fI(t))=−I(t)1+kI(t)[fkI2(t)+(μk+f)I(t)−b(ra+ε)+μ]. $
|
(3.9) |
If $ rb\leq a\mu $, by inequality (3.9), it follows that, for $ t > T $,
$ ˙I(t)≤−I(t)1+kI(t)[(μk+f)I(t)−bε], $
|
which implies
$ lim supt→+∞I(t)≤bεμk+f. $
|
(3.10) |
Since $ I(t)\geq0 $ and inequality (3.10) holds for arbitrary $ \varepsilon > 0 $, we obtain that $ \lim_{t\rightarrow+\infty}I(t) = 0 $. Further, according to the last equation of model (1.3), $ \lim_{t\rightarrow+\infty}u_{2}(t) = 0 $ can be easily obtained.
If $ rb > a\mu $, it follows from inequality (3.9) that, for $ t > T $,
$ \dot{I}(t)\leq \left\{ −fkI(t)1+kI(t)(I(t)−I1(ε))(I(t)−I2(ε)),k>0,−f(I(t)−I2(ε)),k=0. \right. $
|
where
$ I1(ε)=−(μk+f)−√(μk+f)2+4fk(bra+bε−μ)2fk<0,k>0I2(ε)={−(μk+f)+√(μk+f)2+4fk(bra+bε−μ)2fk>0,k>0,b(r+aε)−aμaf,k=0. $
|
Similarly, it follows that
$ lim supt→+∞I(t)≤I2(ε),andlim supt→+∞I(t)≤I2(0)=IM. $
|
(3.11) |
The proof is completed.
For convenience, let us give the following conditions $ (H_1) $, $ (H_2) $ and $ (H_3) $:
$ (H1)c1(e1+2d1)2τ1+c1r2τ3<a.(H2)e12τ1+r2a(a+b+2c1)τ3<e1d1.(H3)rc1b2aτ3<f+μk. $
|
We can obtain the following result.
Theorem 3.4. Assume that $ R_{0}\leq1 $. For any $ \tau_{2}\geq0 $ and $ \tau_{4}\geq0 $, the following statements are true.
(i) If $ rb\leq a\mu $ and conditions $ (H_{1}) $–$ (H_{2}) $ hold, then the disease-free equilibrium $ E_{0} $ is globally attractive in $ X_{1}: = \{\phi\in C^{+}:\; \phi_{1}(0) > 0\} $.
(ii) If $ rb > a\mu $ and conditions $ (H_{1}) $–$ (H_{3}) $ hold, then the disease-free equilibrium $ E_{0} $ is globally attractive in $ X_{1} $.
Proof. It is easy to show that $ X_{1} $ is positively invariant for model (1.3). Let $ (S(t), I(t), u_{1}(t), u_{2}(t)) $ be the solution of model (1.3) with any initial function $ \phi\in X_{1} $. By inequality (2.2), for any sufficiently small $ \varepsilon_{0} > 0 $, there exists a $ t_{1} > 0 $ such that $ S(t) < \frac{r}{a}+\varepsilon_{0}\equiv S_{1}(\varepsilon_{0}) $ for $ t > t_{1} $.
We continue to analyze $ \frac{dV_{1}}{dt} $ given by Eq (3.5). From Eq (3.5) and inequality (3.7), we have that, for $ t\geq 0 $,
$ dV1dt=−a(S(t)−S0)2−μ(1−R0)1+kI(t)I(t)−μk1+kI(t)I2(t)−fI2(t)−c2I(t)u2(t−τ2)−c1e1d1(u1(t)−u01)2+Λ1. $
|
(3.12) |
Note that, for $ t > \tau_1+\tau_3 $, $ \Lambda_{1} $ can be rewritten as
$ Λ1=c1(S(t)−S0)(u1(t)−u1(t−τ1))+c1(u1(t)−u01)(S(t−τ3)−S(t)):=Λ2+Λ3, $
|
where
$ Λ2=c1(S(t)−S0)(u1(t)−u1(t−τ1))=c1(S(t)−S0)∫tt−τ1˙u1(ξ)dξ=c1(S(t)−S0)∫tt−τ1(−e1(u1(ξ)−u01)+d1(S(ξ−τ3)−S0))dξ,Λ3=c1(u1(t)−u01)(S(t−τ3)−S(t))=−c1(u1(t)−u01)∫tt−τ3˙S(ξ)dξ=−c1(u1(t)−u01)∫tt−τ3S(ξ)(a(S0−S(ξ))−bI(ξ)1+kI(ξ)+c1(u01−u1(ξ−τ1)))dξ. $
|
In addition, for $ t > \tau_1+\tau_3 $, we have
$ Λ2≤c1e12∫tt−τ1((S(t)−S0)2+(u1(ξ)−u01)2)dξ+c1d12∫tt−τ1((S(t)−S0)2+(S(ξ−τ3)−S0)2)dξ=c1(e1+d1)2τ1(S(t)−S0)2+c1e12∫tt−τ1(u1(ξ)−u01)2dξ+c1d12∫tt−τ1(S(ξ−τ3)−S0)2dξ. $
|
(3.13) |
Similarly, for $ t > t_{1}+\tau_1+\tau_3 $, we have
$ Λ3≤c1S(ε0)|u1(t)−u01|∫tt−τ3(a|S0−S(ξ)|+bI(ξ)1+kI(ξ)+c1|u01−u1(ξ−τ1)|)dξ≤c1aS(ε0)2∫tt−τ3((u1(t)−u01)2+(S0−S(ξ))2)dξ+c1bS(ε0)2∫tt−τ3((u1(t)−u01)2+I2(ξ)(1+kI(ξ))2)dξ+c21S(ε0)2∫tt−τ3((u1(t)−u01)2+(u01−u1(ξ−τ1))2)dξ=c1S(ε0)2(a+b+c1)τ3(u1(t)−u01)2+c1aS(ε0)2∫tt−τ3(S0−S(ξ))2dξ+c1bS(ε0)2∫tt−τ3I2(ξ)(1+kI(ξ))2dξ+c21S(ε0)2∫tt−τ3(u01−u1(ξ−τ1))2dξ, $
|
(3.14) |
here $ S(t) < S_{1}(\varepsilon_{0}) $ for $ t > t_{1} $ is used. For $ t > t_{1}+\tau_1+\tau_3 $, let us define the following function $ L_2 $,
$ L_{2} = \sum\limits_{i = 1}^{6}V_{i}, $ |
where $ V_1 $ is defined as in the proof of Theorem 3.3,
$ V2=c1aS(ε0)2∫tt−τ3∫tθ(S(ξ)−S0)2dξdθ,V3=c1d12∫tt−τ1∫tθ(S(ξ−τ3)−S0)2dξdθ+c1d1τ12∫tt−τ3(S(ξ)−S0)2dξ,V4=c1bS(ε0)2∫tt−τ3∫tθI2(ξ)(1+kI(ξ))2dξdθ,V5=c1e12∫tt−τ1∫tθ(u1(ξ)−u01)2dξdθ,V6=c21S(ε0)2∫tt−τ3∫tθ(u1(ξ−τ1)−u01)2dξdθ+c21S(ε0)2τ3∫tt−τ1(u1(ξ)−u01)2dξ. $
|
For $ t > t_{1}+\tau_1+\tau_3 $, we calculate the derivatives of $ V_{i} \; (i = 2, \cdots, 6) $ as follows,
$ dV2dt=c1aS(ε0)2[τ3(S(t)−S0)2−∫tt−τ3(S(ξ)−S0)2dξ], $
|
(3.15) |
$ dV3dt=c1d12[τ1(S(t)−S0)2−∫tt−τ1(S(ξ−τ3)−S0)2dξ], $
|
(3.16) |
$ dV4dt=c1bS(ε0)2[τ3I2(t)(1+kI(t))2−∫tt−τ3I2(ξ)(1+kI(ξ))2dξ], $
|
(3.17) |
$ dV5dt=c1e12[τ1(u1(t)−u01)2−∫tt−τ1(u1(ξ)−u01)2dξ], $
|
(3.18) |
$ dV6dt=c21S(ε0)2[τ3(u1(t)−u01)2−∫tt−τ3(u1(ξ−τ1)−u01)2dξ]. $
|
(3.19) |
Combining (3.12)–(3.19), for $ t > t_{1}+\tau_1+\tau_3 $, we finally have
$ dL2dt=6∑i=1dVidt≤−[a−c1(e1+2d1)2τ1−c1aS(ε0)2τ3](S(t)−S0)2−μ(1−R0)1+kI(t)I(t)+c1bS(ε0)2τ3I2(t)(1+kI(t))2−μk1+kI(t)I2(t)−fI2(t)−c2I(t)u2(t−τ2)−[c1e1d1−c1e12τ1−c1S(ε0)2(a+b+2c1)τ3](u1(t)−u01)2. $
|
(3.20) |
Let us show global attractivity of the disease-free equilibrium $ E_{0} $ in the following two cases.
Case (ⅰ) $ rb\leq a\mu $ (which implies $ R_{0} < 1 $) and $ (H_1) $–$ (H_2) $ hold.
From conditions $ (H_{1}) $–$ (H_{2}) $, it has that, for sufficiently small $ \varepsilon_{0} > 0 $, the inequalities
$ a−c1(e1+2d1)2τ1−c1aS(ε0)2τ3>0,e1d1−e12τ1−S(ε0)2(a+b+2c1)τ3>0 $
|
(3.21) |
hold. Furthermore, from Lemma 3.3, we have that $ \lim_{t\rightarrow\infty}I(t) = 0 $ and $ \lim_{t\rightarrow\infty}u_{2}(t) = 0 $. Thus, there exists a $ t_{2} > 0 $ such that, for $ t > t_{2} $,
$ c_{1}bS(\varepsilon_{0})\tau_{3}I(t) \lt \frac{\mu d_{1}c_{1}}{ae_{1}+d_{1}c_{1}}. $ |
In addition, note that
$ 1-R_{0} = 1-\frac{bre_{1}}{\mu(ae_{1}+d_{1}c_{1})} = \frac{\mu(ae_{1}+d_{1}c_{1})-bre_{1}}{\mu(ae_{1}+d_{1}c_{1})} \geq\frac{d_{1}c_{1}}{ae_{1}+d_{1}c_{1}}, $ |
from which we have that, for $ t > t_{2} $,
$ Λ4:=−μ(1−R0)1+kI(t)I(t)+c1bS(ε0)2τ3I2(t)(1+kI(t))2≤−I(t)1+kI(t)[μd1c1ae1+d1c1−c1bS(ε0)2τ3I(t)]≤−μd1c12(ae1+d1c1)I(t)1+kI(t)≤0. $
|
(3.22) |
Hence, it follows from inequalities (3.20)–(3.22) that $ \frac{dL_{2}}{dt}\leq0 $ for $ t > \widetilde{T}: = \max\{t_{1}+\tau_{1}+\tau_3, t_{2}\} $. This indicates that, for $ t > \widetilde{T} $, the function $ L_{2}(t) $ is monotonically decreasing and bounded. Thus, the limitation $ \lim_{t\rightarrow+\infty}L_{2}(t) $ exists.
In addition, by Theorem 2.1, it is not difficult to show that, for $ t > \widetilde{T} $, the second derivative $ L''_2(t) $ is also bounded. This implies that $ L'_{2}(t) $ is uniformly continuous for $ t > \widetilde{T} $. Therefore, it follows from Lemma 3.1 that $ \lim_{t\rightarrow\infty}L'_{2}(t) = 0 $. Again from inequalities (3.20)–(3.22), it follows that
$ \lim\limits_{t\rightarrow\infty}S(t) = S^{0},\; \; \; \; \lim\limits_{t\rightarrow\infty}u_{1}(t) = u_{1}^{0}. $ |
This shows that the disease-free equilibrium $ E_{0} $ is globally attractive.
Case (ⅱ) $ rb > a\mu $ and $ (H_1) $–$ (H_3) $ hold.
Similarly, from condition $ (H_{3}) $, we have that the inequality
$ c1bS(ε0)2τ3<f+μk $
|
(3.23) |
holds for sufficiently small $ \varepsilon_{0} > 0 $. From Lemma 3.3, there exists a $ t_{3} > 0 $ such that $ I(t) < I_M+\varepsilon_{0} $ for $ t > t_{3} $. Then, we have that, for $ t > t_3 $,
$ Λ5:=c1bS(ε0)2τ3I2(t)(1+kI(t))2−μk1+kI(t)I2(t)−fI2(t)=−I2(t)(1+kI(t))2[f(1+kI(t))2+μk(1+kI(t))−c1bS(ε0)2τ3]≤−I2(t)(1+k(IM+ε0))2[f+μk−c1bS(ε0)2τ3]≤0. $
|
(3.24) |
Hence, by inequalities (3.20), (3.21) and (3.24), we can also obtain $ \frac{dL_{2}}{dt}\leq0 $ for $ t > \hat{T}: = \max\{t_{1}+\tau_{1}+\tau_3, t_{3}\} $. By the same arguments as in Case (i), we can obtain
$ \lim\limits_{t\rightarrow\infty}S(t) = S^{0}, \; \; \lim\limits_{t\rightarrow\infty}I(t) = 0, \; \; \lim\limits_{t\rightarrow\infty}u_{1}(t) = u_{1}^{0}. $ |
Furthermore, from the last equation of model (1.3), we can easily have $ \lim_{t\rightarrow\infty}u_{2}(t) = 0 $. This shows that the disease-free equilibrium $ E_{0} $ is globally attractive.
The proof is completed.
From Theorems 3.2 and 3.4, we have the following two corollaries.
Corollary 3.1. Assume that $ R_{0} < 1 $, $ d_{1}c_{1} > ae_{1} $ and $ \tau_{1}+\tau_{3} < \tau_{13}^{0} $. For any $ \tau_{2}\geq0 $ and $ \tau_{4}\geq0 $, the following statements are true.
(i) If $ rb\leq a\mu $ and conditions $ (H_{1})-(H_{2}) $ hold, then the disease-free equilibrium $ E_{0} $ is globally asymptotically stable in $ X_{1} $.
(ii) If $ rb > a\mu $ and conditions $ (H_{1})-(H_{3}) $ hold, then the disease-free equilibrium $ E_{0} $ is globally asymptotically stable in $ X_{1} $.
Corollary 3.2. Assume that $ R_{0} < 1 $ and $ \tau_{1} = \tau_{3} = 0 $. For any $ \tau_{2}\geq0 $ and $ \tau_{4}\geq0 $, then the disease-free equilibrium $ E_{0} $ is globally asymptotically stable in $ X_{1} $.
Remak 3.3. If $ \tau_{i} = 0 \; (i=1,2,3,4
Theoretical analysis of the distribution of the characteristic roots of characteristic equation (3.1) at the endemic equilibrium $ E^{*} = (S^{*}, I^{*}, u_{1}^{*}, u_{2}^{*}) $ usually involves some complicated computations, since there are four time delays $ \tau_{i}\geq0 \; (i=1,2,3,4
(ⅰ) Under what conditions, the time delays $ \tau_{i}\geq0 \; (i=1,2,3,4
(ⅱ) Under what conditions, the time delays $ \tau_{i}\geq0 \; (i=1,2,3,4
In this section, we study the above problems (i)–(ii) by constructing suitable Lyapunov functionals.
For convenience, let us denote the following conditions,
$ (H4)τ1=τ2=τ3=τ4=0.(H5)d1c1≤ae1,τ1≥0,τ3≥0,τ2=τ4=0.(H6)d2c2≤fe2,τ2≥0,τ4≥0,τ1=τ3=0.(H7)d1c1≤ae1,d2c2≤fe2,τ1≥0,τ2≥0,τ3≥0,τ4≥0. $
|
For problem (ⅰ) above, we have the following result.
Theorem 4.1. Assume that $ R_{0} > 1 $. If one of conditions $ (H_4) $–$ (H_7) $ holds, then the endemic equilibrium $ E^{*} $ is globally asymptotically stable in $ X_{2}: = \{\phi\in C^{+}: \phi_{i}(0) > 0, \; i = 1, 2\} $.
Proof. It is easy to show that the set $ X_{2} $ is positively invariant for model (1.3). Since $ E^{*} $ is the equilibrium of model (1.3), the following equalities hold,
$ r−aS∗−bI∗1+kI∗−c1u∗1=0,bS∗1+kI∗−μ−fI∗−c2u∗2=0,−e1u∗1+d1S∗=0,−e2u∗2+d2I∗=0. $
|
(4.1) |
Define a Lyapunov functional $ W_{1} $ on $ X_{2} $ as follows,
$ W1=(1+kI∗)(ϕ1(0)−S∗−S∗lnϕ1(0)S∗)+ϕ2(0)−I∗−I∗lnϕ2(0)I∗+c1(1+kI∗)2d1(ϕ3(0)−u∗1)2+c22d2(ϕ4(0)−u∗2)2. $
|
It is clear that $ W_{1} $ is continuous on $ X_{2} $ and positive definite with respect to $ E^{*} $.
Calculating the derivative of $ W_{1} $ along any solution $ (S(t), I(t), u_{1}(t), u_{2}(t)) $ of model (1.3), it follows that, for $ t\geq0 $,
$ dW1dt=(1+kI∗)(S(t)−S∗)[a(S∗−S(t))+bI∗1+kI∗−bI(t)1+kI(t)+c1(u∗1−u1(t−τ1))]+(I(t)−I∗)[bS(t)1+kI(t)−bS∗1+kI(t)+bS∗1+kI(t)−bS∗1+kI∗+f(I∗−I(t))+c2(u∗2−u2(t−τ2))]+c1(1+kI∗)d1(u1(t)−u∗1)[−e1(u1(t)−u∗1)+d1(S(t−τ3)−S∗)]+c2d2(u2(t)−u∗2)[−e2(u2(t)−u∗2)+d2(I(t−τ4)−I∗)], $
|
here Eq (4.1) is used. Note that
$ (1+kI^{*})(S(t)-S^{*})\left(\frac{bI^{*}}{1+kI^{*}}-\frac{bI(t)}{1+kI(t)}\right) +(I(t)-I^{*})\left(\frac{bS(t)}{1+kI(t)}-\frac{bS^{*}}{1+kI(t)}\right) = 0, $ |
from which we have that, for $ t\geq0 $,
$ dW1dt=−a(1+kI∗)(S(t)−S∗)2−[f+bkS∗(1+kI(t))(1+kI∗)](I(t)−I∗)2−c1e1(1+kI∗)d1(u1(t)−u∗1)2−c2e2d2(u2(t)−u∗2)2+(1+kI∗)Υ1+Π1, $
|
(4.2) |
where
$ Υ1=c1(S(t)−S∗)(u∗1−u1(t−τ1))+c1(S(t−τ3)−S∗)(u1(t)−u∗1),Π1=c2(I(t)−I∗)(u∗2−u2(t−τ2))+c2(I(t−τ4)−I∗)(u2(t)−u∗2). $
|
We consider global asymptotic stability of the endemic equilibrium $ E^{*} $ in the following four cases.
If condition $ (H_4) $ holds, it has that $ \Upsilon_{1} = \Pi_{1} = 0 $ and $ \frac{dW_{1}}{dt} $ is negative definite with respect to $ E^{*} $. Hence, the endemic equilibrium $ E^{*} $ is globally asymptotically stable (see, for example, [29,30]).
If condition $ (H_5) $ holds, we have that $ \Pi_{1} = 0 $. Let us consider another functional as follows,
$ W_{2} = W_{1}+\frac{a(1+kI^{*})}{2}\int_{-\tau_{3}}^{0}(\phi_1(\xi)-S^{*})^2d\xi +\frac{c_1e_{1}(1+kI^{*})}{2d_1} \int_{-\tau_{1}}^{0}\left(\phi_3(\xi)-u_{1}^{*}\right)^{2}d\xi. $ |
$ W_{2} $ is continuous on $ X_{2} $, positive definite with respect to $ E^{*} $, and satisfies condition (ii) of Lemma 3.1 in [35] on $ \partial X_{2} = \overline{X_{2}}\setminus X_{2} $.
Calculating the derivative of $ W_{2} $ along any solution $ (S(t), I(t), u_{1}(t), u_{2}(t)) $ of model (1.3), it follows that, for $ t\geq0 $,
$ dW2dt=dW1dt+a(1+kI∗)2[(S(t)−S∗)2−(S(t−τ3)−S∗)2]+c1e1(1+kI∗)2d1[(u1(t)−u∗1)2−(u1(t−τ1)−u∗1)2]. $
|
(4.3) |
By Eqs (4.2) and (4.3), and the following inequality of arithmetic and geometric means:
$ Υ1≤√d1c1ae1[a2(S(t)−S∗)2+c1e12d1(u1(t−τ1)−u∗1)2+a2(S(t−τ3)−S∗)2+c1e12d1(u1(t)−u∗1)2], $
|
(4.4) |
we have that, for $ t\geq0 $,
$ dW2dt≤−a(1+kI∗)2(1−√d1c1ae1)[(S(t)−S∗)2+(S(t−τ3)−S∗)2]−c1e1(1+kI∗)2d1(1−√d1c1ae1)[(u1(t)−u∗1)2+(u1(t−τ1)−u∗1)2]−[f+bkS∗(1+kI(t))(1+kI∗)](I(t)−I∗)2−c2e2d2(u2(t)−u∗2)2. $
|
It follows from condition $ (H_{5}) $ that $ \frac{dW_{2}}{dt}\leq0 $ for $ t\geq0 $. Hence, the endemic equilibrium $ E^{*} $ is stable. Furthermore, $ \frac{dW_{3}}{dt} = 0 $ implies $ I(t) = I^{*} $ and $ u_{2}(t) = u_{2}^{*} $.
Let $ M_{2} $ be the largest invariant set in the set
$ \Gamma_{2}: = \left\{\phi\in \overline{X}_{2}:\; W_{2} \lt \infty \; \text{and}\; \frac{dW_{2}}{dt} = 0\right\}. $ |
Then, it follows that
$ \Gamma_{2} \subset \{\phi\in X_{2}: \phi_{2}(0) = I^{*},\; \phi_{4}(0) = u_{2}^{*}\}. $ |
From model (1.3) and the invariance of $ M_{2} $, we can easily get that $ M_{2} = \{E^{*}\} $. Thus, it follows from Lemma 3.1 in [35] that the endemic equilibrium $ E^{*} $ is globally asymptotically stable.
If condition $ (H_6) $ holds, it follows that $ \Upsilon_{1} = 0 $. Let us consider a functional as follows,
$ W_{3} = W_{1} +\frac{f}{2}\int_{-\tau_{4}}^{0}\left(\phi_2(\xi)-I^{*}\right)^{2}d\xi +\frac{c_2e_{2}}{2d_2}\int_{-\tau_{2}}^{0}\left(\phi_4(\xi)-u_{2}^{*}\right)^{2}d\xi. $ |
$ W_{3} $ is continuous on $ X_{2} $, positive definite with respect to $ E^{*} $, and satisfies condition (ii) of Lemma 3.1 in [35] on $ \partial X_{2} = \overline{X_{2}}\setminus X_{2} $.
Calculating the derivative of $ W_{3} $ along any solution $ (S(t), I(t), u_{1}(t), u_{2}(t)) $ of model (1.3), it follows that, for $ t\geq0 $,
$ dW3dt=dW1dt+f2[(I(t)−I∗)2−(I(t−τ4)−I∗)2]+c2e22d2[(u2(t)−u∗2)2−(u2(t−τ2)−u∗2)2]. $
|
(4.5) |
By Eqs (4.2) and (4.5), and the following inequality of arithmetic and geometric means:
$ Π1≤√d2c2fe2[f2(I(t)−I∗)2+c2e22d2(u2(t−τ2)−u∗2)2+f2(I(t−τ4)−I∗)2+c2e22d2(u2(t)−u∗2)2], $
|
(4.6) |
we have that, for $ t\geq0 $,
$ dW3dt≤−a(1+kI∗)(S(t)−S∗)2−c1e1(1+kI∗)d1(u1(t)−u∗1)2−f2(1−√d2c2fe2)[(I(t)−I∗)2+(I(t−τ4)−I∗)2]−bkS∗(1+kI(t))(1+kI∗)(I(t)−I∗)2−c2e22d2(1−√d2c2fe2)[(u2(t)−u∗2)2+(u2(t−τ2)−u∗2)2]. $
|
It follows from condition $ (H_{6}) $ that $ \frac{dW_{3}}{dt}\leq0 $ for $ t\geq0 $. Furthermore, $ \frac{dW_{3}}{dt} = 0 $ implies $ S(t) = S^{*} $ and $ u_{1}(t) = u_{1}^{*} $. By the same arguments as in the situation of condition $ (H_5) $, we can also show that the endemic equilibrium $ E^{*} $ is globally asymptotically stable.
If condition $ (H_7) $ holds, let us consider the following Lyapunov functional,
$ W4=W1+a(1+kI∗)2∫0−τ3(ϕ1(ξ)−S∗)2dξ+f2∫0−τ4(ϕ2(ξ)−I∗)2dξ+c1e1(1+kI∗)2d1∫0−τ1(ϕ3(ξ)−u∗1)2dξ+c2e22d2∫0−τ2(ϕ4(ξ)−u∗2)2dξ. $
|
$ W_{4} $ is continuous on $ X_{2} $, positive definite with respect to $ E^{*} $, and satisfies condition (ii) of Lemma 3.1 in [35] on $ \partial X_{2} = \overline{X_{2}}\setminus X_{2} $.
Calculating the derivative of $ W_{4} $ along any solution $ (S(t), I(t), u_{1}(t), u_{2}(t)) $ of model (1.3), it follows that, for $ t\geq0 $,
$ dW4dt=dW1dt+a(1+kI∗)2[(S(t)−S∗)2−(S(t−τ3)−S∗)2]+f2[(I(t)−I∗)2−(I(t−τ4)−I∗)2]+c1e1(1+kI∗)2d1[(u1(t)−u∗1)2−(u1(t−τ1)−u∗1)2]+c2e22d2[(u2(t)−u∗2)2−(u2(t−τ2)−u∗2)2]. $
|
Further, by Eq (4.2) and inequalities (4.4) and (4.6), we have that, for $ t\geq0 $,
$ dW4dt≤−a(1+kI∗)2(1−√d1c1ae1)[(S(t)−S∗)2+(S(t−τ3)−S∗)2]−f2(1−√d2c2fe2)[(I(t)−I∗)2+(I(t−τ4)−I∗)2]−bkS∗(1+kI(t))(1+kI∗)(I(t)−I∗)2−c1e1(1+kI∗)2d1(1−√d1c1ae1)[(u1(t)−u∗1)2+(u1(t−τ1)−u∗1)2]−c2e22d2(1−√d2c2fe2)[(u2(t)−u∗2)2+(u2(t−τ2)−u∗2)2]. $
|
It follows from condition $ (H_{7}) $ that $ \frac{dW_{4}}{dt}\leq0 $ for $ t\geq0 $. Furthermore, if $ d_{1}c_{1} < ae_{1} $, then $ \frac{dW_{4}}{dt} = 0 $ implies that $ S(t) = S^{*} $ and $ u_{1}(t) = u_{1}^{*} $. By the same arguments as in the situation of condition $ (H_5) $, we can show that the endemic equilibrium $ E^{*} $ is globally asymptotically stable. If $ d_{1}c_{1} = ae_{1} $, also by the same arguments as in the situation of $ d_{1}c_{1} = ae_{1} $ in Theorem 3.3, we can show that $ E^{*} $ is globally asymptotically stable.
The proof is completed.
Remak 4.1. In the situation of condition $ (H_7) $, Theorem 4.1 indicates that the time delays $ \tau_{i}\geq0 \; (i = 1, 2, 3, 4) $ are harmless for global asymptotic stability of the endemic equilibrium $ E^{*} $.
Now, let us consider problem (ⅱ). Note that $ R_{0} = \frac{bre_{1}}{\mu(ae_{1}+d_{1}c_{1})} > 1 $ implies $ rb > a\mu $. Let us denote the following conditions,
$ (H8)c1(e1+2d1)2τ1+c1r2τ3+c2bIM2(1+kI∗)(1+kIM)τ4<a.(H9)c2(e2+2d2)2τ2+c1br2aτ3+c22[fIM+bkS∗IM(1+kI∗)(1+kIM)]τ4<f+bkS∗(1+kI∗)(1+kIM).(H10)e12τ1+r2a(a+b1+kI∗+2c1)τ3<e1d1.(H11)e22τ2+12[bIM1+kIM+fIM+bkS∗IM(1+kI∗)(1+kIM)+2c2IM]τ4<e2d2. $
|
In conditions $ (H_8) $–$ (H_{11}) $ above, the definition of $ I_{M} $ is given in Lemma 3.3 (ⅱ). We have the following result.
Theorem 4.2. Assume that $ R_{0} > 1 $. If conditions $ (H_{8}) $–$ (H_{11}) $ hold, then the endemic equilibrium $ E^{*} $ is globally attractive in $ X_{2} $.
Proof. Let $ (S(t), I(t), u_{1}(t), u_{2}(t)) $ be the solution of model (1.3) with any initial function $ \phi\in X_{2} $. From Theorem 2.1 and Lemma 3.3, for sufficient small $ \varepsilon_1 > 0 $, there exists a $ T_{1} > 0 $ such that, for $ t > T_{1} $,
$ S(t) \lt \frac{r}{a}+\varepsilon_{1}: = S_{1}(\varepsilon_{1}), \; \; I(t) \lt I_{M}+\varepsilon_{1}: = I_{M}(\varepsilon_{1}). $ |
Hence, from Eq (4.2), it has that, for $ t > T_{1} $,
$ dW1dt≤−a(1+kI∗)(S(t)−S∗)2−[f+bkS∗(1+kIM(ε1))(1+kI∗)](I(t)−I∗)2−c1e1(1+kI∗)d1(u1(t)−u∗1)2−c2e2d2(u2(t)−u∗2)2+(1+kI∗)|Υ1|+|Π1|. $
|
(4.7) |
For simplicity, denote
$ A(\varepsilon_{1}): = \left(f+ \frac{bkS^{*}} {(1+kI^{*})(1+kI_{M}(\varepsilon_{1}))}\right)I_{M}(\varepsilon_{1}). $ |
Note that $ \Upsilon_{1} $ and $ \Pi_{1} $ can be rewritten as
$ Υ1=c1(S(t)−S∗)(u1(t)−u1(t−τ1))+c1(S(t−τ3)−S(t))(u1(t)−u∗1):=Υ2+Υ3,Π1=c2(I(t)−I∗)(u2(t)−u2(t−τ2))+c2(I(t−τ4)−I(t))(u2(t)−u∗2):=Π2+Π3. $
|
Let us give appropriate estimations on $ |\Upsilon_{2}| $, $ |\Upsilon_{3}| $, $ |\Pi_{2}| $ and $ |\Pi_{3}| $.
It follows that, $ t > \tau_1+\tau_2+\tau_3+\tau_4+T_1 $,
$ |Υ2|=|c1(S(t)−S∗)∫tt−τ1˙u1(ξ)dξ|=|c1(S(t)−S∗)∫tt−τ1(−e1(u1(ξ)−u∗1)+d1(S(ξ−τ3)−S∗))dξ|≤c1e12∫tt−τ1((S(t)−S∗)2+(u1(ξ)−u∗1)2)dξ+c1d12∫tt−τ1((S(t)−S∗)2+(S(ξ−τ3)−S∗)2)dξ=c1(e1+d1)2τ1(S(t)−S∗)2+c1e12∫tt−τ1(u1(ξ)−u∗1)2dξ+c1d12∫tt−τ1(S(ξ−τ3)−S∗)2dξ, $
|
(4.8) |
$ |Υ3|=|−c1(u1(t)−u∗1)∫tt−τ3˙S(ξ)dξ|=|c1(u1(t)−u∗1)∫tt−τ3S(ξ)[a(S∗−S(ξ))+bI∗1+kI∗−bI(ξ)1+kI(ξ)+c1(u∗1−u1(ξ−τ1))]dξ|=|c1(u1(t)−u∗1)∫tt−τ3S(ξ)[a(S∗−S(ξ))+b(I∗−I(ξ))(1+kI∗)(1+kI(ξ))+c1(u∗1−u1(ξ−τ1))]dξ| $
|
$ ≤c1S1(ε1)|u1(t)−u∗1|∫tt−τ3(a|S∗−S(ξ)|+b1+kI∗|I∗−I(ξ)|+c1|u∗1−u1(ξ−τ1)|)dξ≤c1aS1(ε1)2∫tt−τ3((u1(t)−u∗1)2+(S∗−S(ξ))2)dξ+c1bS1(ε1)2(1+kI∗)∫tt−τ3((u1(t)−u∗1)2+(I∗−I(ξ))2)dξ+c21S1(ε1)2∫tt−τ3((u1(t)−u∗1)2+(u∗1−u1(ξ−τ1))2)dξ=c1S1(ε1)2[a+b1+kI∗+c1]τ3(u1(t)−u∗1)2+c1aS1(ε1)2∫tt−τ3(S∗−S(ξ))2dξ+c1bS1(ε1)2(1+kI∗)∫tt−τ3(I∗−I(ξ))2dξ+c21S1(ε1)2∫tt−τ3(u∗1−u1(ξ−τ1))2dξ, $
|
(4.9) |
$ |Π2|=|c2(I(t)−I∗)∫tt−τ2˙u2(ξ)dξ|=|c2(I(t)−I∗)∫tt−τ2(−e2(u2(ξ)−u∗2)+d2(I(ξ−τ4)−I∗))dξ|≤c2e22∫tt−τ2((I(t)−I∗)2+(u2(ξ)−u∗2)2)dξ+c2d22∫tt−τ2((I(t)−I∗)2+(I(ξ−τ4)−I∗)2)dξ≤c2(e2+d2)2τ2(I(t)−I∗)2+c2e22∫tt−τ2(u2(ξ)−u∗2)2dξ+c2d22∫tt−τ2(I(ξ−τ4)−I∗)2dξ. $
|
(4.10) |
$ |Π3|=|−c2(u2(t)−u∗2)∫tt−τ4˙I(ξ)dξ|=|c2(u2(t)−u∗2)∫tt−τ4I(ξ)[bS(ξ)1+kI(ξ)−bS∗1+kI∗+f(I∗−I(ξ))+c2(u∗2−u2(ξ−τ2))]dξ|=|c2(u2(t)−u∗2)∫tt−τ4[bI(ξ)1+kI(ξ)(S(ξ)−S∗)+bkS∗I(ξ)(1+kI∗)(1+kI(ξ))(I∗−I(ξ))+fI(ξ)(I∗−I(ξ))+c2I(ξ)(u∗2−u2(ξ−τ2))]dξ|≤c2|u2(t)−u∗2|∫tt−τ4[bIM(ε1)1+kIM(ε1)|S(ξ)−S∗|+A(ε1)|I∗−I(ξ)|+c2IM(ε1)|u∗2−u2(ξ−τ2)|]dξ≤c2bIM(ε1)2(1+kIM(ε1))∫tt−τ4((u2(t)−u∗2)2+(S(ξ)−S∗)2)dξ+c22A(ε1)∫tt−τ4((u2(t)−u∗2)2+(I(ξ)−I∗)2)dξ+c22IM(ε1)2∫tt−τ4((u2(t)−u∗2)2+(u2(ξ−τ2)−u∗2)2)dξ=c22[bIM(ε1)1+kIM(ε1)+A(ε1)+c2IM(ε1)]τ4(u2(t)−u∗2)2+c2bIM(ε1)2(1+kIM(ε1))∫tt−τ4(S(ξ)−S∗)2dξ+c22A(ε1)∫tt−τ4(I(ξ)−I∗)2dξ+c22IM(ε1)2∫tt−τ4(u2(ξ−τ2)−u∗2)2dξ. $
|
(4.11) |
For $ t > \tau_1+\tau_2+\tau_3+\tau_4+T_{1} $, let us define the following function,
$ U = W_{1}+(1+kI^{*})U_1+U_2, $ |
where
$ U1=c1d12[∫tt−τ1∫tθ(S(ξ−τ3)−S∗)2dξdθ+τ1∫tt−τ3(S(ξ)−S∗)2dξ]+c1aS1(ε1)2∫tt−τ3∫tθ(S(ξ)−S∗)2dξdθ+c1bS1(ε1)2(1+kI∗)∫tt−τ3∫tθ(I∗−I(ξ))2dξdθ,+c21S1(ε1)2[∫tt−τ3∫tθ(u1(ξ−τ1)−u∗1)2dξdθ+τ3∫tt−τ1(u1(ξ)−u∗1)2dξ]+c1e12∫tt−τ1∫tθ(u1(ξ)−u∗1)2dξdθ, $
|
$ U2=c2d22[∫tt−τ2∫tθ(I(ξ−τ4)−I∗)2dξdθ+τ2∫tt−τ4(I(ξ)−I∗)2dξ]+c22A(ε1)∫tt−τ4∫tθ(I(ξ)−I∗)2dξdθ+c2bIM(ε1)2(1+kIM(ε1))∫tt−τ4∫tθ(S(ξ)−S∗)2dξdθ+c22IM(ε1)2[∫tt−τ4∫tθ(u2(ξ−τ2)−u∗2)2dξdθ+τ4∫tt−τ2(u2(ξ)−u∗2)2dξ]+c2e22∫tt−τ2∫tθ(u2(ξ)−u∗2)2dξdθ. $
|
Computing the derivatives of $ U_1 $ and $ U_2 $, we easily get that, for $ t > \tau_1+\tau_2+\tau_3+\tau_4+T_{1} $,
$ dU1dt=[c1d12τ1+c1aS1(ε1)2τ3](S(t)−S∗)2−c1d12∫tt−τ1(S(ξ−τ3)−S∗)2dξ−c1aS1(ε1)2∫tt−τ3(S(ξ)−S∗)2dξ+c1bS1(ε1)2(1+kI∗)τ3(I(t)−I∗)2−c1bS1(ε1)2(1+kI∗)∫tt−τ3(I(ξ)−I∗)2dξ+[c1e12τ1+c21S1(ε1)2τ3](u1(t)−u∗1)2−c21S1(ε1)2∫tt−τ3(u1(ξ−τ1)−u∗1)2dξ−c1e12∫tt−τ1(u1(ξ)−u∗1)2dξ, $
|
(4.12) |
$ dU2dt=c2bIM(ε1)2(1+kIM(ε1))τ4(S(t)−S∗)2−c2bIM(ε1)2(1+kIM(ε1))∫tt−τ4(S(ξ)−S∗)2dξ+[c2d22τ2+c22A(ε1)τ4](I(t)−I∗)2−c2d22∫tt−τ2(I(ξ−τ4)−I∗)2dξ−c22A(ε1)∫tt−τ4(I(ξ)−I∗)2dξ+[c2e22τ2+c22IM(ε1)2τ4](u2(t)−u∗2)2−c22IM(ε1)2∫tt−τ4(u2(ξ−τ2)−u∗2)2dξ−c2e22∫tt−τ2(u2(ξ)−u∗2)2dξ. $
|
(4.13) |
In summary, by (4.7)–(4.13), we have that, for $ t > \tau_1+\tau_2+\tau_3+\tau_4+T_{1} $,
$ dUdt=dW1dt+(1+kI∗)dU1dt+dU2dt≤−(1+kI∗)[a−(2d1+e1)c12τ1−c1aS1(ε1)2τ3−c2bIM(ε1)2(1+kI∗)(1+kIM(ε1))τ4](S(t)−S∗)2−[f+bkS∗(1+kIM(ε1))(1+kI∗)−(2d2+e2)c22τ2−c1bS1(ε1)2τ3−c22A(ε1)τ4](I(t)−I∗)2−(1+kI∗)c1[e1d1−e12τ1−S1(ε1)2(a+b1+kI∗+2c1)τ3](u1(t)−u∗1)2−c2[e2d2−e22τ2−12(bIM(ε1)1+kIM(ε1)+A(ε1)+2c2IM(ε1))τ4](u2(t)−u∗2)2:=−(1+kI∗)Q1(ε1)(S(t)−S∗)2−Q2(ε1)(I(t)−I∗)2−(1+kI∗)c1Q3(ε1)(u1(t)−u∗1)2−c2Q4(ε1)(u2(t)−u∗2)2. $
|
(4.14) |
Furthermore, from conditions $ (H_8) $–$ (H_{11}) $, we see that, for sufficiently small $ \varepsilon_{1} > 0 $, we have that $ Q_i(\varepsilon_{1}) > 0 \; (i = 1, 2, 3, 4) $. This shows that $ \frac{dU}{dt}\leq0 $ for $ t > \tau_1+\tau_2+\tau_3+\tau_4+T_{1} $. By similar arguments as in the proof of Theorem 3.4, we can have
$ \lim\limits_{t\rightarrow+\infty}S(t) = S^{*}, \; \; \lim\limits_{t\rightarrow+\infty}I(t) = I^{*}, \; \; \lim\limits_{t\rightarrow+\infty}u_{1}(t) = u_{1}^{*}, \; \; \lim\limits_{t\rightarrow+\infty}u_{2}(t) = u_{2}^{*}. $ |
This proves that the endemic equilibrium $ E^{*} $ is globally attractive.
The proof is completed.
Remak 4.2. If $ \tau_{i} = 0 \; (i=1,2,3,4
In this paper, we consider the SI epidemic model (1.3) with two feedback control variables and four time delays. In biology, model (1.3) has more general biological significance. Then, by skillfully constructing appropriate Lyapunov functionals, and combining Lyapunov–LaSalle invariance principle and Barbalat's lemma, some sufficient conditions for global dynamics of the equilibria of model (1.3) are established. In the case of $ d_{1}c_{1}\leq ae_{1} $, Theorem 3.3 gives complete conclusion on global asymptotic stability of the disease-free equilibrium $ E_{0} $. In the case of $ d_{1}c_{1} > ae_{1} $, in Theorem 3.4, global attractivity of the disease-free equilibrium $ E_{0} $ is considered under conditions $ (H_1) $–$ (H_3) $. Note that, in the case, it has from Theorem 3.2 that local asymptotic stability of the disease-free equilibrium $ E_{0} $ also depends on the time delays $ \tau_1 $ and $ \tau_3 $. Hence, The set of conditions $ (H_1) $–$ (H_3) $ has certain rationality. Furthermore, as a special case, Theorems 3.2, 3.3 and 3.4 improve and generalize Theorem 1 in [28] and Theorem 2.1 in [17].
We also establish some sufficient conditions for global dynamics of the endemic equilibrium $ E^{*} $ of model (1.3). Theorem 4.1 shows that, if condition $ (H_5) $, or $ (H_6) $, or $ (H_7) $ holds, the time delays $ \tau_1 $ and $ \tau_3 $, or $ \tau_2 $ and $ \tau_4 $, or $ \tau_i \; (i = 1, 2, 3, 4) $ are harmless for global asymptotic stability of the endemic equilibrium $ E^{*} $ of model (1.3). If condition $ (H_4) $ holds, i.e., $ \tau_i = 0 \; (i = 1, 2, 3, 4) $, we see that Theorem 4.1 includes Theorem 2 in [28] and Theorem 2.2 in [17] as a special case. Furthermore, in Theorem 4.2, under condition $ R_0 > 1 $, a set of sufficient conditions $ (H_8) $–$ (H_{11}) $ is obtained to ensure global attractivity of the endemic equilibrium $ E^{*} $ of model (1.3). Note that the subsequent numerical simulations imply that any one of time delays $ \tau_i \; (i = 1, 2, 3, 4) $ may destroy local asymptotic stability of the endemic equilibrium $ E^{*} $ of model (1.3) and result in the occurrence of periodic oscillations etc.. Hence, in the set of conditions $ (H_8) $–$ (H_{11}) $, it should be feasible to have some certain limits on the lengths of time delays $ \tau_i \; (i = 1, 2, 3, 4) $.
In the following, let us give some numerical simulations to summarize the applications of Theorem 3.4, Corollary 3.1 and Theorem 4.2.
Firstly, let us choose the values of a set of parameters as follows,
$ r=1.2,a=1,b=0.4,k=1,c1=0.8,μ=0.25,f=1,c2=1,e1=0.6,d1=1.2,e2=1,d2=1. $
|
(5.1) |
By computations, we have that $ 0.96 = d_1c_1 > ae_1 = 0.6 $, $ 0.48 = rb > a\mu = 0.25 $, $ R_{0}\approx0.738 < 1 $, $ E_{0} = (0.462, 0, 0.923, 0) $ and $ \tau_{13}^{0}\approx4.542 $. Furthermore, conditions $ (H_1) $–$ (H_3) $ become the following inequalities,
$ (\hat{H})\; \; \; 1.2\tau_1+0.48\tau_3 \lt 1,\; \; \; 0.3\tau_1+1.8\tau_3 \lt 0.5. $ |
It has from Theorem 3.2 that the disease-free equilibrium $ E_{0} $ is locally asymptotically stable for $ \tau_{1}+\tau_{3} < \tau_{13}^{0}\approx4.542 $ and unstable for $ \tau_{1}+\tau_{3} > \tau_{13}^{0}\approx4.542 $. If time delays $ \tau_{1} $ and $ \tau_{3} $ satisfy more restrictive condition $ (\hat{H}) $, it has from Theorem 3.4 or Corollary 3.1 that the disease-free equilibrium $ E_{0} $ is also globally asymptotically stable.
Let us choose $ \tau_1 = 0.75 $ and $ \tau_{3} = 0.15 $. We see that conditions $ \tau_{1}+\tau_{3} < \tau_{13}^{0}\approx4.542 $ and $ (\hat{H}) $ are satisfied. Figure 1(a) shows that the disease-free equilibrium $ E_{0} $ is globally asymptotically stable.
Let us choose $ \tau_1 = 2 $ and $ \tau_{3} = 1.25 $. We see that condition $ (\hat{H}) $ does not hold, but condition $ \tau_{1}+\tau_{3} < \tau_{13}^{0}\approx4.542 $ is still satisfied. Figure 1(b) shows that the disease-free equilibrium $ E_{0} $ is locally asymptotically stable.
Let us further choose $ \tau_1 = 2.75 $ and $ \tau_{3} = 2 $. We see that condition $ \tau_{1}+\tau_{3} > \tau_{13}^{0}\approx4.542 $ holds. Figure 1(c) shows that the disease-free equilibrium $ E_{0} $ becomes unstable and periodic oscillations occur. In Figure 1(a)–(c), for simplicity, $ \tau_2 $ and $ \tau_4 $ are fixed as $ \tau_2 = 3 $ and $ \tau_4 = 6 $, respectively.
Secondly, let us give numerical simulations in the situation of the basic reproduction number $ R_{0} > 1 $.
Let us choose the values of set of parameters as follows,
$ r=2,a=0.8,b=1,k=1.5,c1=1.1,μ=0.25,f=0.8,c2=0.8,e1=0.8,d1=1,e2=0.5,d2=1.8. $
|
(5.2) |
By computations, we have that $ R_{0}\approx3.678 > 1 $, $ E^{*}\approx(0.870, 0.130, \; 1.087, 0.467) $. Furthermore, conditions $ (H_8) $–$ (H_{11}) $ approximately become the following inequalities,
$ (\tilde{H})\; \; \; \left\{ 1.54τ1+1.1τ3+0.132τ4<0.8,1.64τ2+1.375τ3+0.481τ4<1.246,0.4τ1+4.796τ3<0.8,0.25τ2+1.570τ4<0.278. \right. $
|
If time delays $ \tau_i \; (i = 1, 2, 3, 4) $ satisfy condition $ (\tilde{H}) $, it has from Theorem 4.2 that the endemic equilibrium $ E^{*} $ is globally attractive.
Let us choose $ \tau_1 = 0.4 $, $ \tau_{2} = 0.35 $, $ \tau_3 = 0.1 $ and $ \tau_{4} = 0.12 $. We see that condition $ (\tilde{H}) $ is satisfied. Figure 2(a) shows that the endemic equilibrium $ E^{*} $ is globally attractive.
Let us choose larger values here, $ \tau_1 = 1.6 $, $ \tau_{2} = 1.4 $, $ \tau_3 = 0.4 $ and $ \tau_{4} = 0.48 $. We see that condition $ (\tilde{H}) $ does not hold. Figure 2(b) show that the endemic equilibrium $ E^{*} $ may be still attractive.
Let us further choose $ \tau_1 = 2 $, $ \tau_{2} = 1.75 $, $ \tau_3 = 0.6 $ and $ \tau_{4} = 0.8 $. We see that condition $ (\tilde{H}) $ does not hold. Figure 2(c) shows that the endemic equilibrium $ E^{*} $ becomes unstable and periodic oscillations occur.
Moreover, Figure 3(a)–(d) show that any one of time delays $ \tau_{i} $ ($ i = 1, 2, 3, 4 $) can destroy local asymptotic stability of the endemic equilibrium $ E^{*} $ of model (1.3). Here, the values of the parameters of model (1.3) are the same as Eq (5.2).
At the end of the paper, in view of Figure 1(b) and Figure 2(b) above, we would like to point out that conditions $ (H_1) $–$ (H_3) $ in Theorem 3.3 and conditions $ (H_8) $–$ (H_{11}) $ in Theorem 4.2 are actually conservative and worth of further improving. In addition, for global asymptotic stability of the endemic equilibrium $ E^{*} $ of model (1.3), in the case of $ d_{1}c_{1} > a_{1}e_{1} $ or $ d_{2}c_{2} > f e_{2} $, to give some sufficient conditions which are different from conditions $ (H_{8}) $–$ (H_{11}) $ may be also interesting, since Figure 4(a)–(d) show that model (1.3) may have richer dynamic behaviors. Here, the values of the parameters of model (1.3) are also the same as Eq (5.2). Further, it would be interesting to extend model (1.3) to the case of non-autonomous model and to consider the uniform persistence and existence of almost periodic solutions etc. [38,39].
This paper is supported by Beijing Natural Science Foundation (No.1202019) and National Natural Science Foundation of China (No.11971055). The authors would like to thank the reviewers and the editor for their careful reading, helpful comments and suggestions that greatly improved the paper.
All authors declare no conflicts of interest in this paper.
[1] |
Hofmann SG, Asnaani A, Vonk JJ, et al. (2012) The efficacy of cognitive behavioral therapy: A review of meta-analyses. Cognitive Ther Res 36: 427-440. doi: 10.1007/s10608-012-9476-1
![]() |
[2] |
Hofmann SG, Smits JA (2008) Cognitive-behavioral therapy for adult anxiety disorders: a meta-analysis of randomized placebo-controlled trials. J Clin Psychiatry 69: 621-632. doi: 10.4088/JCP.v69n0415
![]() |
[3] | Hofmann SG, Otto MW, Pollack MH, et al. (2015) D-Cycloserine Augmentation of Cognitive Behavioral Therapy for Anxiety Disorders: an Update. Curr Psychiatry Rep 17(1): 1-5. |
[4] |
Hofmann SG (2008) Cognitive processes during fear acquisition and extinction in animals and humans: Implications for exposure therapy of anxiety disorders. Clin Psychol Rev 28: 199-210. doi: 10.1016/j.cpr.2007.04.009
![]() |
[5] |
Singewald N, Schmuckermair C, Whittle N, et al. (2015) Pharmacology of cognitive enhancers for exposure—based therapy for fear, anxiety, and trauma-related disorder. Pharmacol Ther 149:150-190. doi: 10.1016/j.pharmthera.2014.12.004
![]() |
[6] |
Hofmann SG, Meuret AE, Smits JA, et al. (2006) Augmentation of exposure therapy with D-cycloserine for social anxiety disorder. Arch Gen Psychiatry 63: 298-304. doi: 10.1001/archpsyc.63.3.298
![]() |
[7] |
Smits JA, Rosenfield D, Davis ML, et al. (2014) Yohimbine enhancement of exposure therapy for social anxiety disorder: A randomized controlled trial. Biol Psychiatry 75: 840-846. doi: 10.1016/j.biopsych.2013.10.008
![]() |
[8] |
Davis M, Ressler K, Rothbaum BO, et al. (2006) Effects of D-cycloserine on extinction: translation from preclinical to clinical work. Biol Psychiatry 60: 369-375. doi: 10.1016/j.biopsych.2006.03.084
![]() |
[9] |
Richardson R, Ledgerwood L, Cranney J (2004) Facilitation of fear extinction by D-cycloserine: theoretical and clinical implications. Learn Mem 11: 510-516. doi: 10.1101/lm.78204
![]() |
[10] |
Bouton ME, Vurbic D, Woods AM (2008) D-cycloserine facilitates context-specific fear extinction learning. Neurobiol Learn Mem 90: 504-510. doi: 10.1016/j.nlm.2008.07.003
![]() |
[11] |
Ressler KJ, Rothbaum BO, Tannenbaum L, et al. (2004) Cognitive enhancers as adjuncts to psychotherapy: Use of D-cycloserine in phobic individuals to facilitate extinction of fear. Arch Gen Psychiatry 61: 1136-1144. doi: 10.1001/archpsyc.61.11.1136
![]() |
[12] |
Otto MW, Tolin DF, Simon NM, et al. (2010) Efficacy of D-cycloserine for enhancing response to cognitive-behavior therapy for panic disorder. Biol Psychiatry 67: 365-370. doi: 10.1016/j.biopsych.2009.07.036
![]() |
[13] |
Hofmann SG (2014) D-cycloserine for treating anxiety disorders: making good exposures better and bad exposures worse. Depress Anxiety 31: 175-177. doi: 10.1002/da.22257
![]() |
[14] |
Hofmann SG, Hüweler R, Mackillop J, et al. (2012) Effects of d-cycloserine on craving to alcohol cues in problem drinkers: Preliminary findings. Am J Drug Alcohol Abuse 38: 101-107. doi: 10.3109/00952990.2011.600396
![]() |
[15] |
Lee JL, Milton AL, Everitt BJ (2006) Reconsolidation and extinction of conditioned fear: inhibition and potentiation. J Neurosci 26: 10051-10056. doi: 10.1523/JNEUROSCI.2466-06.2006
![]() |
[16] |
Hofmann SG, Smits JA, Rosenfield D, et al. (2013) D-Cycloserine as an augmentation strategy with cognitive-behavioral therapy for social anxiety disorder. Am J Psychiatry 170: 751-758. doi: 10.1176/appi.ajp.2013.12070974
![]() |
[17] |
Smits JAJ, Rosenfield D, Otto MW, et al. (2013) D-cycloserine enhancement of exposure therapy for social anxiety disorder depends on the success of exposure sessions. J Psychiat Res 47: 1455-1461. doi: 10.1016/j.jpsychires.2013.06.020
![]() |
[18] |
Holmes A, Quirk GJ (2010) Pharmacological facilitation of fear extinction and the search for adjunct treatments for anxiety disorders—the case of yohimbine. Trends Pharmacol Sci 31: 2-7. doi: 10.1016/j.tips.2009.10.003
![]() |
[19] |
Cain CK, Blouin AM, Barad M (2004). Adrenergic transmission facilitates extinction of conditional fear in mice. Learn Mem 11: 179-187. doi: 10.1101/lm.71504
![]() |
[20] |
O'Carroll RE, Drysdale E, Cahill L, et al. (1999) Stimulation of the noradrenergic system enhances and blockade reduces memory for emotional material in man. Psychol Med 29: 1083-1088. doi: 10.1017/S0033291799008703
![]() |
[21] |
Powers MB, Smits JAJ, Otto MW, et al. (2009) Facilitation of fear extinction in phobic participants with a novel cognitive enhancer: A randomized placebo controlled trial of yohimbine augmentation. J Anxiety Disord 23: 350-356. doi: 10.1016/j.janxdis.2009.01.001
![]() |
[22] |
Lupien SJ, McEwen BS, Gunnar MR, et al. (2009) Effects of stress throughout the lifespan on the brain, behavior and cognition. Nat Rev Neurosci 10: 434-445. doi: 10.1038/nrn2639
![]() |
[23] | De Quervain DJF, Aerni A, Schelling G, et al. (2009) Glucocorticoids and the regulation of memory in health and disease. J Epidemiol commu H 30(3): 358-370. |
[24] | Roozendaal B, McGaugh JL (1997) Glucocorticoid receptor agonist and antagonist administration into the basolateral but not central amygdala modulates memory storage, Neurobiol Learn Mem 67: 176-179. |
[25] | Lupien SJ, Fiocco A, Wan N, et al. (2005) Stress hormones and human memory function across the lifespan. Psychoneuroendocrinol 30(3): 225-242. |
[26] | Otto MW, McHugh Rk, Kantak KM (2010) Combined pharmacotherapy and cognitive-behavioral therapy for anxiety disorders: Medication effects, glucocorticoids, and attenuated treatment outcomes. Clin Psychol 17(2): 91-103. |
[27] | Andreano JM, Cahill L (2006) Glucocorticoid release and memory consolidation in men and women. Psychol Sci 17(6): 466-470. |
[28] | Roozendaal B, Williams CL, McGaugh JL (1999) Glucocorticoid receptor activation in the rat nucleus of the solitary tract facilitates memory consolidation: Involvement of the basolateral amygdala. Eur J Neurosci 11(4): 1317-1323. |
[29] | Cai WH, Blundell J, Han J, et al. (2006) Postreactivation glucocorticoids impair recall of established fear memory. J Neurosci 26(37): 9560-9566. |
[30] | Pakdel R, Rashidy-Pour A (2007) Microinjections of the dopamine D2 receptor antagonist sulpiride into the medial prefrontal cortex attenuate glucocorticoid-induced impairment of long-term memory retrieval in rats. Neurobiol Learn Mem 87(3): 385-390. |
[31] | Barrett D, Gonzalez-Lima F (2004) Behavioral effects of metyrapone on Pavlovian extinction. Neurosci Lett 371(2-3): 91-96. |
[32] | Bohus B, Lissak K (1968) Adrenocortical hormones and avoidance behavior of rats. Int J Neuropharmacol 7(4): 301-306. |
[33] | Yang YL, Chao PK, Lu KT (2006) Systemic and intra-amygdala administration of glucocorticoid agonist and antagonist modulate extinction of conditioned fear. Neuropsychopharmacol 31(5): 912-924. |
[34] |
Lass-Hennemann J, Michael T (2014) Endogenous cortisol levels influence exposure therapy in spider phobia. Behav Res Ther 60: 39-45. doi: 10.1016/j.brat.2014.06.009
![]() |
[35] | Soravia LM, Heinrichs M, Aerni A, et al. (2006) Glucocorticoids reduce phobic fear in humans. Proc Natl Acad Sci USA 103(14): 5585-5590. |
[36] |
Soravia LM, Heinrichs M, Winzeler L, et al. (2014) Glucocorticoids enhance in vivo exposure-based therapy if spider phobia. Depress Anxiety 31: 429-435. doi: 10.1002/da.22219
![]() |
[37] | De Quervain DJ, Bentz D, Michael T, et al. (2011) Glucocorticoids enhance extinction-based psychotherapy. Proc Natl Acad Sci USA 108(16): 6621-6625. |
[38] |
Meuret AE, Trueba AF, Abelson JL, et al. (2015) High cortisol awakening response and cortisol levels moderate exposure-based psychotherapy success. Psychoneuroendocrinol 51: 331-340. doi: 10.1016/j.psyneuen.2014.10.008
![]() |
[39] | Siegmund A, Koster L, Meves AM, et al. (2011) Stress hormones during flooding therapy and their relationship to therapy outcome in patients with panic disorder and agoraphobia. J Psychiatr Res 45(3): 339-346. |
[40] | Suris A, North C, Adinoff B, et al. (2010) Effects of exogenous glucocorticoid on combat-related PTSD symptoms. Ann Clin Psychiatry 22: 274-279. |
[41] | Aerni A, Traber R, Hock C, et al. (2004) Low-dose cortisol for symptoms of posttraumatic stress disorder. Am J Psychiatry 161(8): 1488-1490. |
[42] | Fries E, Hellhammer DH, Hellhammer J (2006) Attenuation of the hypothalamic-pituitary-adrenal axis responsivity to the Trier Social Stress Test by the benzodiazepine alprazolam. Psychoneuroendocrinol 31(10): 1278-1288. |
[43] | Pomara N, Willoughby LM, Sidtis JJ, et al. (2005) Cortisol response to diazepam: Its relationship to age, dose, duration of treatment, and presence of generalized anxiety disorder. Psychopharmacol 178(1): 1-8. |
[44] |
Brown RM, Crane AM, Goldman PS (1979) Regional distribution of monoamines in the cerebral cortex and subcortical structures of rhesus monkey: concentrations and in vivo synthesis. Brain Res 168: 133-150. doi: 10.1016/0006-8993(79)90132-X
![]() |
[45] | Goldman-Rakic PS (1991) Prefrontal cortical dysfunction in schizophrenia: the relevance of working memory. In: Psychopathology and the Brain, edited by B. Carroll, New York: Raven, 1-23. |
[46] | Sawaguchi T, Goldman-Rakic PS (1994) The role of D1-dopamine receptor in working memory: Local injections of dopamine antagonists into the prefrontal cortex of rhesus monkeys performing an oculomotor delayed-response task. J Neurophysiol 71(2): 515-528. |
[47] | Seamans JK, Yang CR (2004) The principal features and mechanisms of dopamine modulation in the prefrontal cortex. Prog Neurobiol 74(1): 1-58. |
[48] | Bromberg-Martin ES, Matsumoto M, Hikosaka O (2010) Dopamine in motivational control: rewarding, aversive, and alerting. Neuron 68(5): 815-834. |
[49] | Nikolaus S, Antke C, Beu M, et al. (2010) Cortical GABA, striatal dopamine and midbrain serotonin as the key players in compulsive and anxiety disorders: Results from in vivo imaging studies. Rev Neurosci 21(2): 119-139. |
[50] | Olver JS, O'Keefe G, Jones GR, et al. (2009) Dopamine D1 receptor binding in the striatum of patients with obsessive-compulsive disorder. J Affect Disord 114(1-3): 321-326. |
[51] | De la Mora MP, Gallegos-Cari A, Arizmendi-Garcia Y, et al. (2010) Role of dopamine receptor mechanisms in the amygdaloid modulation of fear and anxiety: Structural and functional analysis. Prog Neurobiol 90(2): 198-216. |
[52] | Koo MS, Kim EJ, Roh D, et al. (2010) Role of dopamine in the pathophysiology and treatment of obsessive-compulsive disorder. Expert Rev Neurother 10(2): 275-290. |
[53] | Insel TR (2010) The challenge of translation in social neuroscience: A review of oxytocin, vasopressin, and affiliative behavior. Neuron 65(6): 768-779. |
[54] | Kosfeld M, Heinrichs M, Zak PJ, et al. (2005) Oxytocin increases trust in humans. Nat 435(7042): 673-676. |
[55] |
Meyer-Lindenberg A, Domes G, Kirsch P, et al. (2011). Oxytocin and vasopressin in the human brain: Social neuropeptides for translational medicine. Nat Rev Neurosci 12(9): 524-538. doi: 10.1038/nrn3044
![]() |
[56] |
Frith CD (2008) Social Cognition. Philos Trans R Soc Biol Sci, 363: 2033-2039. doi: 10.1098/rstb.2008.0005
![]() |
[57] |
Eisenberg N, Miller PA (1987) The relation of empathy to prosocial and related behaviors. Psycholog Bull, 101: 91-119. doi: 10.1037/0033-2909.101.1.91
![]() |
[58] |
Perez-Rodriguez MM, Mahon K, Russo M, et al. (2015) Oxytocin and social cognition in affective and psychotic disorders. Eur Neuropsychopharmacol 25: 265-282. doi: 10.1016/j.euroneuro.2014.07.012
![]() |
[59] | Zak PJ, Kurzban R & Matzner WT (2005) Oxytocin is associated with human trustworthiness. Horm Behav 48(5): 522-527. |
[60] | Grewen KM, Girdler SS, Amico J, et al. (2005) Effects of partner support on resting oxytocin, cortisol, norepinephrine, and blood pressure before and after warm partner contact. Psychosom Med 67(4): 531-538. |
[61] | Scantamburlo G, Hansenne M, Fuchs S, et al. (2007). Plasma oxytocin levels and anxiety in patients with major depression. Psychoneuroendocrinol 32(4): 407-410. |
[62] | Goldman M, Marlow-O'Connor M, Torres I, et al. (2008) Diminished plasma oxytocin in schizophrenic patients with neuroendocrine dysfunction and emotional deficits. Schizophr Res 98(1-3): 247-255. |
[63] | Green L, Fein D, Modahl C, et al. (2001) Oxytocin and autistic disorder: Alterations in peptide forms. Biol Psychiatry 50(8): 609-613. |
[64] | Cyranowski JM, Hofkens TL, Frank E, et al. (2008) Evidence of dysregulated peripheral oxytocin release among depressed women. Psychosom Med 70(9): 967-975. |
[65] | Taylor SE, Gonzaga GC, Klein LC, et al. (2006) Relation of oxytocin to psychological stress responses and hypothalamic-pituitary-adrenocortical axis activity in older women. Psychosom Med 68(2): 238-245. |
[66] | Hoge EA, Pollack MH, Kaufman RE, et al. (2008) Oxytocin levels in social anxiety disorder. CNS Neurosci Ther 14(3): 165-170. |
[67] | Born J, Lange T, Kern W, et al. (2002) Sniffing neuropeptides: A transnasal approach to the human brain. Nat Neurosci 5(6): 514-516. |
[68] | Mikolajczak M, Gross JJ, Lane A, et al. (2010) Oxytocin makes people trusting, not gullible. Psychol Sci 21(8): 1072-1074. |
[69] | Mikolajczak M, Pinon N, Lane A, et al. (2010) Oxytocin not only increases trust when money is at stake, but also when confidential information is in the balance. Biol Psychol 85(1): 182-184. |
[70] | Baumgartner T, Heinrichs M, Vonlanthen A, et al. (2008) Oxytocin shapes the neural circuitry of trust and trust adaptation in humans. Neuron 58(4): 639-650. |
[71] | Hofmann S G, Fang A, Brager D N (in press). Effect of intranasal oxytocin administration on psychiatric symptoms: A meta-analysis of placebo-controlled studies.Psychiatry Res. |
[72] | Guastella AJ, Howard AL, Dadds MR, et al. (2009) A randomized controlled trial of intranasal oxytocin as an adjunct to exposure therapy for social anxiety disorder. Psychoneuroendocrinol 34(6): 917-923. |
[73] | MacDonald E, Dadds MR, Brennan JL, et al. (2011) A review of safety, side-effects and subjective reactions to intranasal oxytocin in human research. Psychoneuroendocrinol 36(8): 1114-1126. |
[74] | Labuschagne I, Phan KL, Wood A, et al. (2010) Oxytocin attenuates amygdala reactivity to fear in generalized social anxiety disorder. Neuropsychopharmacol 35(12): 2403-2413. |
[75] |
Labuschagne I, Phan KL, Wood A, et al. (2012) Medial frontal hyperactivity to sad faces in generalized social anxiety disorder and modulation by oxytocin. Int J Neuropsychopharmacol 15: 883-896. doi: 10.1017/S1461145711001489
![]() |
[76] | Fang A, Hoge E A, Heinrichs M, et al. (2014) Attachment Style Moderates the Effects of Oxytocin on Social Behaviors and Cognitions During Social Rejection: Applying an RDoC Framework to Social Anxiety. Clin Psychol Sci 2 (6):740-747. |
[77] | Minzenberg MJ, Carter CS (2008) Modafinil: A review of neurochemical actions and effects on cognition. Neuropsychopharmacol 33(7): 1477-1502. |
[78] | Volkow ND, Fowler JS, Logan J, et al. (2009) Effects of modafinil on dopamine and dopamine transporters in the male human brain: clinical implications. JAMA 301(11): 1148-1154. |
[79] |
Madras BK, Xie Z, Lin Z, et al. (2006) Modafinil occupies dopamine and norepinephrine transporters in vivo and modulates the transporters and trace amine activity in vitro. J Pharmacol Exp Ther 319: 561-569. doi: 10.1124/jpet.106.106583
![]() |
[80] | Hermant JF, Rambert FA, Duteil J (1991) Awakening properties of modafinil: Effect on nocturnal activity in monkeys (Macacamulatta) after acute and repeated administration. Psychopharmacol 103(1): 28-32. |
[81] | Kahbazi M, Ghoreishi A, Rahiminejad F, et al. (2009) A randomized, double-blind and placebo-controlled trial of modafinil in children and adolescents with attention deficit and hyperactivity disorder. Psychiatry Res 168(3): 234-237. |
[82] | Randall DC, Shneerson JM, Plaha KK, et al. (2003) Modafinil affects mood, but not cognitive function, in healthy young volunteers. Hum Psychopharmacol 18(3): 163-173. |
[83] | Wong YN, King SP, Simcoe D, et al. (1999) Open-label, single-dose pharmacokinetic study of modafinil tablets: Influence of age and gender in normal subjects. J Clin Pharmacol 39(3): 281-288. |
[84] |
Murphy HM, Ekstrand D, Tarchick M, et al. (2015) Modafinil as a cognitive enhancer of spatial working memory in rats. Physiol Behav 142: 126-130. doi: 10.1016/j.physbeh.2015.02.003
![]() |
[85] | Rasetti R, Mattay VS, Stankevich B, et al. (2010) Modulatory effects of modafinil on neural circuits regulating emotion and cognition. Neuropsychopharmacol 35(10): 2101-2109. |
[86] | Schwartz JR, Hirshkowitz M, Erman MK, et al. (2003) Modafinil as adjunct therapy for daytime sleepiness in obstructive sleep apnea: a 12-week, open-label study. Chest 124(6): 2192-2199. |
[87] | Zifko UA, Rupp M, Schwarz S, et al. (2002) Modafinil in treatment of fatigue in multiple sclerosis. Results of an open-label study. J Neurol 249(8): 983-987. |
[88] |
Balanza-Martinez V, Fries GR, Colpo GD, et al. (2011) Therapeutic use of omega-3 fatty acids in bipolar disorder. Expert Rev Neurotherapeutics 11: 1029-1047. doi: 10.1586/ern.11.42
![]() |
[89] |
Bloch MH, Qawasmi A (2011) Omega-3 fatty acid supplementation for the treatment of children with attention-deficit/hyperactivity disorder symptomatology: Systematic review and meta-analysis. J Am Acad Child Adolesc Psychiatry 50: 991-1000. doi: 10.1016/j.jaac.2011.06.008
![]() |
[90] | Cosci F, Abrams K, Schruers KRJ, et al. (2006) Effect of nicotine on 35% CO2-induced anxiety: A study in healthy volunteers. Nicotine Tob Res 8, 511-517. |
[91] |
Gertsik L, Poland RE, Bresee C, et al. (2012) Omega-3 fatty acid augmentation of citalopram treatment for patients with major depressive disorder. J Clin Psychopharmacol 32: 61-64. doi: 10.1097/JCP.0b013e31823f3b5f
![]() |
[92] |
Lesperance FO, Frasure-Smith N, St-Andre E, et al. (2011) The efficacy of omega-3 supplementation for major depression: A randomized controlled trial. J Clin Psychiatry 72: 1054-1062. doi: 10.4088/JCP.10m05966blu
![]() |
[93] | Masdrakis VG, Papakostas YG, Vaidakis N, et al. (2008) Caffeine challenge in patients with panic disorder: Baseline differences between those who panic and those who do not. Depress Anxiety 25, E72-E79. |
[94] |
Mystkowski JL, Mineka S, Vernon LL, et al. (2003) Changes in caffeine states enhance return of fear in spider phobia. J Consult Clin Psychol 71: 243-250. doi: 10.1037/0022-006X.71.2.243
![]() |
[95] | Salin-Pascual RJ, Basanez-Villa E (2003) Changes in compulsion and anxiety symptoms with nicotine transdermal patches in non-smoking obsessive-compulsive disorder patients. Revista de Investigacion Clinica 55: 650-654. |
[96] | Culver NC, Vervliet B, Craske MG (2014). Compound Extinction Using the Rescorla–Wagner Model to Maximize Exposure Therapy Effects for Anxiety Disorders. Clin Psychol Sci 2167702614542103. |
[97] |
McNamara RK, Carlson SE (2006) Role of omega-3 fatty acids in brain development and function: potential implications for the pathogenesis and prevention of psychopathology. Prostaglandins Leukot Essent Fatty Acids 75: 329-349. doi: 10.1016/j.plefa.2006.07.010
![]() |
[98] | Timonen M, Horrobin D, Jokelainen J, et al. (2004) Fish consumption and depression: The Northern Finland 1966 birth cohort study. J Affective Disord 82: 447-452. |
[99] |
McNamara RK (2011) Omega-3 fatty acid deficiency: A preventable risk factor for schizophrenia? Schizophr Res 129: 215-216. doi: 10.1016/j.schres.2010.12.017
![]() |
[100] | Hofmann SG, Sawyer AT, Korte KJ, et al. (2009) Is it beneficial to add pharmacotherapy to cognitive-behavioral therapy when treating anxiety disorders? A meta-analytic review. Int J Cogn Ther 2: 160-175. |
1. | Manuel De la Sen, Asier Ibeas, Santiago Alonso-Quesada, On the Supervision of a Saturated SIR Epidemic Model with Four Joint Control Actions for a Drastic Reduction in the Infection and the Susceptibility through Time, 2022, 19, 1660-4601, 1512, 10.3390/ijerph19031512 | |
2. | Ke Guo, Wanbiao Ma, Global dynamics of a delayed air pollution dynamic model with saturated functional response and backward bifurcation, 2022, 21, 1534-0392, 3831, 10.3934/cpaa.2022124 | |
3. | Meng Li, Ke Guo, Wanbiao Ma, Uniform Persistence and Global Attractivity in a Delayed Virus Dynamic Model with Apoptosis and Both Virus-to-Cell and Cell-to-Cell Infections, 2022, 10, 2227-7390, 975, 10.3390/math10060975 | |
4. | Qing Yang, Xinhong Zhang, Daqing Jiang, Asymptotic behavior of a stochastic SIR model with general incidence rate and nonlinear Lévy jumps, 2022, 107, 0924-090X, 2975, 10.1007/s11071-021-07095-7 | |
5. | Karrar Qahtan Al-Jubouri, Raid Kamel Naji, Arvind Kumar Misra, Dynamics Analysis of a Delayed Crimean‐Congo Hemorrhagic Fever Virus Model in Humans, 2024, 2024, 1110-757X, 10.1155/2024/4818840 | |
6. | Luyao Zhao, Mou Li, Wanbiao Ma, Hopf bifurcation and stability analysis of a delay differential equation model for biodegradation of a class of microcystins, 2024, 9, 2473-6988, 18440, 10.3934/math.2024899 |