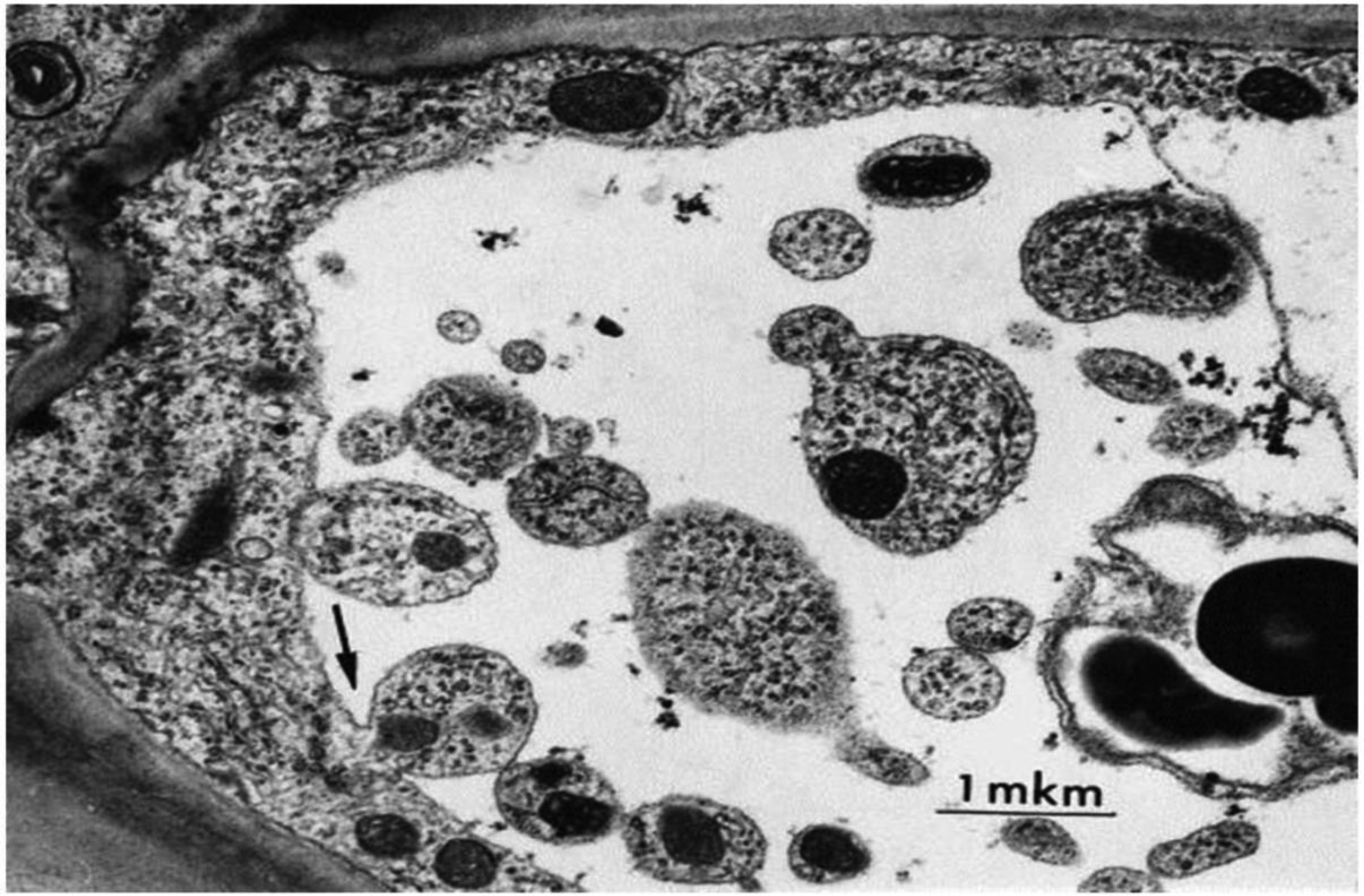
Citation: Larisa I. Fedoreyeva, Boris F. Vanyushin. Possible existence of a system similar to bacterial restriction–modification in plants[J]. AIMS Molecular Science, 2020, 7(4): 396-413. doi: 10.3934/molsci.2020020
[1] | Sarmishta Mukhopadhyay, Sayak Ganguli, Santanu Chakrabarti . Shigella pathogenesis: molecular and computational insights. AIMS Molecular Science, 2020, 7(2): 99-121. doi: 10.3934/molsci.2020007 |
[2] | Jehad Shaikhali, Gunnar Wingsle . Redox-regulated transcription in plants: Emerging concepts. AIMS Molecular Science, 2017, 4(3): 301-338. doi: 10.3934/molsci.2017.3.301 |
[3] | Mohammad Ahmad Ahmad Odah . Unlocking the genetic code: Exploring the potential of DNA barcoding for biodiversity assessment. AIMS Molecular Science, 2023, 10(4): 263-294. doi: 10.3934/molsci.2023016 |
[4] | Fumiaki Uchiumi, Akira Sato, Masashi Asai, Sei-ichi Tanuma . An NAD+ dependent/sensitive transcription system: Toward a novel anti-cancer therapy. AIMS Molecular Science, 2020, 7(1): 12-28. doi: 10.3934/molsci.2020002 |
[5] | Jean Emmanuel Mbosso Teinkela, Philippe Belle Ebanda Kedi, Jean Baptiste Hzounda Fokou, Michelle Isaacs, Lisette Pulchérie Yoyo Ngando, Gaelle Wea Tchepnou, Hassan Oumarou, Xavier Siwe Noundou . In vitro anti-trypanosomal activity of crude extract and fractions of Trichoscypha acuminata stem bark, Spathodea campanulata flowers, and Ficus elastica lianas on Trypanosoma brucei brucei. AIMS Molecular Science, 2024, 11(1): 63-71. doi: 10.3934/molsci.2024005 |
[6] | Praveen Kumar Neela, Rajeshwari B.V, Mahamad Irfanulla Khan, Shahistha Parveen Dasnadi, Gosla Srinivas Reddy, Akhter Husain, Vasavi Mohan . Genetic influences on non-syndromic cleft lip palate: The impact of BMP4, RUNX2, PAX7, and TGFB3 allelic variations. AIMS Molecular Science, 2024, 11(4): 322-329. doi: 10.3934/molsci.2024019 |
[7] | Oscar Cienfuegos-Jiménez, Abril Morales-Hernández, Olivia A. Robles-Rodríguez, Sergio Bustos-Montes, Kevin A. Bañuelos-Alduncin, Aurora R. Cortés-Castillo, Hugo D. Barreto-Hurtado, Luis Carrete-Salgado, Iván A. Marino-Martínez . High-yield production and purification of the fusion pH-responsive peptide GST-pHLIP in Escherichia coli BL21. AIMS Molecular Science, 2022, 9(4): 136-144. doi: 10.3934/molsci.2022008 |
[8] | Isabella Martins Lourenço, Amedea Barozzi Seabra, Marcelo Lizama Vera, Nicolás Hoffmann, Olga Rubilar Araneda, Leonardo Bardehle Parra . Synthesis and application of zinc oxide nanoparticles in Pieris brassicae larvae as a possible pesticide effect. AIMS Molecular Science, 2024, 11(4): 351-366. doi: 10.3934/molsci.2024021 |
[9] | Yutaka Takihara, Ryuji Otani, Takuro Ishii, Shunsuke Takaoka, Yuki Nakano, Kaori Inoue, Steven Larsen, Yoko Ogino, Masashi Asai, Sei-ichi Tanuma, Fumiaki Uchiumi . Characterization of the human IDH1 gene promoter. AIMS Molecular Science, 2023, 10(3): 186-204. doi: 10.3934/molsci.2023013 |
[10] | Jin Hwan Do . Genome-wide transcriptional comparison of MPP+ treated human neuroblastoma cells with the state space model. AIMS Molecular Science, 2015, 2(4): 440-460. doi: 10.3934/molsci.2015.4.440 |
Restriction enzymes are commonly found in bacteria, where they help ward off attacks by foreign DNA from viruses, particularly bacteriophages [1],[2]. Unlike higher organisms, in which the recognition and destruction of viruses, bacteria, and other pathogens occur extracellularly, in bacteria, protection against foreign DNA (DNA of plants and animals in which they live) occurs intracellularly (i.e., when foreign DNA penetrates the cytoplasm of bacteria). Bacteria have also evolved the ability to “label” their own DNA with methylating bases on certain sequences. Recognition sites in bacterial DNA are modified by enzymes called DNA methyltransferases, which transfer a methyl group to S-adenosyl-L-methionine (SAM), thereby protecting DNA from the action of nucleases [3]. Methylation occurs either at adenine or cytosine, resulting in the formation of N6-methyladenine (m6A), N4-methylcytosine (m4C), or C5-methylcytosine (m5C). Methylation prevents restriction enzymes from cutting the genome at restriction sites. However, the genomes of viruses in which the restriction sites are not modified can be cut by restriction enzymes and destroyed. The combination of methylation and restriction is called the restriction–modification system (R-M) [4].
The molecular basis of R-M of DNA was first described in 1962 [5]. Host specificity was explained in molecular terms as an endonucleolytic cleavage of foreign DNA, where cellular DNA is protected from restriction by modification of adenosyl or cytosyl bases within defined recognition sites. Thus, R-M systems are composed of pairs of opposing enzyme activities: a restriction endonuclease (REase) and a DNA methyltransferase (MTase). R-M systems are currently classified into four groups, based on their molecular structure, sequence recognition, cleavage position, and cofactor requirements [6].
The type I R-M system consists of three subunits: S for sequence recognition, M for modification, and R for restriction [7]–[9]. The S and M subunits include DNA methyltransferase, which recognizes and modifies DNA within a specific sequence and shows a preference for DNA hemimethylation Type I enzymes nonspecifically cleave DNA at a considerable distance from an unmethylated site. The type II system is the simplest and most common R-M system, consisting of MTase and restriction enzyme activities. Type II restriction endonucleases, due to their unique specificity, are an indispensable tool in DNA recombination technology, which are of great use in both science and medicine. They are also used as model systems for studying aspects of DNA-protein interactions and specific mechanisms of Mg2+-dependent phosphodiester hydrolysis [10].
The type III system can be combined with the type I system as one family of ATP-dependent restriction enzymes [11]. However, they are similar in mechanism to type I enzymes [12].
Finally, type IV enzymes recognize modified DNA with low sequence selectivity. It is believed that modification-dependent type IV enzymes arose as a result of a competitive coevolutionary interaction between phages and their hosts [2],[13],[14]; in other words, the hosts used the restriction blocked by the modification to protect against phage infection. The weak sequence selectivity of the Type IV systems might simply reflect the lack of endogenous targets for the enzyme. Selection would act to spare any co-resident MTases. This differs from Type II enzymes, where the MTase and REase must coevolve to allow the host to survive. Each Type IV system is compatible with some suite of Type I–III MTases.
In addition to R-M systems, DNA can also be methylated via orphan MTases. Orphan MTases methylate DNA at site-specific sequences and do not have a related endonuclease [15],[16]. In bacteria, the two best studied orphan MTases are Escherichia coli DNA adenosine methyltransferase (Dam) and methyltransferase regulated by the Caulobacter crescentus cell cycle (CcrM) [15],[16]. Orphan Mtases, which are not associated with true restriction endonucleases, help in self-defense against invasive DNA along with restriction endonucleases.
Cytosine DNA methylation in plants is more rich and diverse compared with animals [17],[18]. These enzymes in plants, as well as in animals, are homologous to bacterial DMT. It is carried out by the families of specific enzymes that belong to at least three classes of DMTs: DNA methyltransferase (MET) and chromomethyltransferase (CMT) [19]. Plant genomes have three types of methylation sites CG, CNG, and CNN (where N = C, T, or A [20],[21]. Methylation of previously unmethylated DNA de novo is carried out by the DMT family, called domains rearranged methyltransferases (DRMs).
Until now, the role of adenine methylation in eukaryotes has not been determined. m6A was found in the genome of the nematode and genes encoding adenine DNA methyltransferase were found [22]. Gene orthologs are found in the genomes of many eukaryotes, including plants [17].
A previous study identified numerous cytoplasmic regions of various sizes surrounded by a closed membrane and containing one or several mitochondria, fragments of membranes of the rough endoplasmic reticulum, and polyribosomal complexes in vacuoles of coleoptile cells of wheat seedlings [23]. These were isolated cytoplasmic structures not associated with the main cytoplasm of the cell, and the cytoplasm in the detected vesicles had no signs of destruction, in contrast to the contents of the surrounding vacuole.
Other studies have identified two endonucleases (WEN1 and WEN2) and an adenine DNA methyltransferase (WAD) in the vesicular fraction of aging etiolated wheat coleoptiles [24]–[26]. In this study, we investigated the specificity of enzymes in this system and consider the possible existence of such an R-M system in plants.
Seeds of the winter wheat Triticum aestivum L cultivar Mironovskaya 808 were grown in the light and in the dark at 26°C on wet filter paper placed in plastic cuvettes immersed in water, which was changed daily [23].
Coleoptiles of 7 day-old wheat seedlings were homogenized at 4°C in a homogenization buffer (50 mM Tris-HCl, pH 7.5, 0.4 M sucrose, 1 mg/ml BSA; 5 ml buffer/g tissue). The homogenate was filtered through four layers of gauze and one layer of Miracloth. The nuclei from the homogenate were separated by centrifugation at 600 g for 15 min. Then, the supernatant was centrifuged at 3000 g for 15 min, precipitating the vesicular fraction [27]. After the separation of the vesicular fraction of mitochondria, free mitochondria were obtained by the centrifugation of the supernatant at 8000 g for 15 min. Sediments (fractions) of nuclei, vesicles and free mitochondria were washed with homogenization buffer without BSA. The purification of cell compartments was carried out by ultracentrifugation in a sucrose gradient. The purity and integrity of the organelles were tested by microscopy.
The electron microscopy investigation of the isolated subcellular particle fractions and serial sections of coleoptile was carried out by standard procedures described earlier [23].
The protein fraction containing nuclease activity was extracted from the vesicular fraction of 7 day-old coleoptiles with a buffer containing 50 mM Tris-HCl, pH 7.5, 0.8 M sucrose and 0.35 M NaCl. The purification of enzymes was carried out according to the method presented in [24],[25]. Protein concentration was determined spectrophotometrically with the Nanophotometer Implen by absorption at λ = 280 nm and by the Bradford method [28]. The analysis of protein preparations and determination of molecular masses of proteins were carried out by electrophoresis in 12.5% SDS-PAGE according to the Laemmli method [29].
The substrates used for the endonucleases WEN1 and WEN2 DNA λ phage were purchased from Fermentas (Lithuania); unlike unmethylated DNA (dcm−, dam−), methylated (dcm+, dam+) DNA of λ phage contains 5-methylcytosine residues in the Cm5CHGG sequences and N6-methyladenine residues in the Gm6ATC sites. RNA and DNA were thymic and were purchased from Fermentas (Lithuania). DNA hydrolysis products were analyzed in horizontal electrophoresis in 1.2% agarose.
Adenine DNA methyltransferase was also isolated from the vesicular fraction of mitochondria from coleoptiles of 7 day-old wheat seedlings by extraction with a buffer containing 0.4 M sucrose, 5 mM EDTA, 1 mM dithiothreitol, 1 mg/ml bovine serum albumin and 20 mM Tris-HCl pH 7.5 as described in [26].
The methylation of DNA was carried out in the presence of 3H–SAM (S-adenosyl-L-methionine) [26]. For DNA methylation, in order to isolate nucleic bases, 1 µl of a solution (0.2 mg/ml) of BSA was added to 100 µg of thymic DNA; then, 20 µl of the analyzed protein solution was added to 3H–SAM and the mixture was incubated at 37°C for 2–3 hours. The reaction was stopped by adding cold alcohol in a ratio of 1:3. DNA was applied to a plate with a thin layer of cellulose (10 g per 100 ml water). Chromatography was performed in a butanol–water–ammonia system in a ratio of 60:10:0.1 to separate free 3Н–SAM.
Methylation of λ phage (dcm−, dam−) 5-cytosine methyltransferase SssI (Thermo Scientific), which methylates the 5′-CpG-3′ site, was carried out according to the standard procedure. Methylation of λ phage (dcm−, dam−) at N6-adenine was carried out by WAD-DNA methyltransferase, which methylates the 5′-TGm6ATCA-3′ site, as described above using non-radioactive SAM.
Nuclease activity was determined spectrophotometrically at 260 nm using thymic DNA (A260 nm = 1.0 in 0.5M acetate buffer, pH 5.0). The reaction was carried out at 37°C for 1 h. An amount of enzyme was taken as a unit of activity that caused an increase in the optical density of the solution by 0.01 units under standard incubation conditions. In addition to thymus DNA, λ phage DNA [(dcm−, dam−) and (dcm+, dam+)] was used as a substrate.
The nuclease activity was also determined in 12.5% polyacrylamide gel with fused thymic DNA (0.2 mg/ml) [30].
To 7 µl of 10 mM Tris-HCL buffer pH 7.5, containing 2 µg of unmethylated DNA λ phage (dcm−, dam−) and methylated by WAD methylase DNA (dam+) or by cytosine methyltransferase SssI DNA (dcm+), 1 µl of wheat endonucleases WEN1 or WEN2 was added. The mixture was incubated at 37°C for 2 h. The hydrolysis products were analyzed in horizontal electrophoresis in 1.2% agarose.
5-carboxyfluorescein (FAM) oligos have been synthesized in various contexts containing either a CG site or a CNG site and FAM oligos containing methylcytosine at the CG and CNG sites were synthesized at Syntol (Russia).
Synthetic oligos (1 µg) were hydrolyzed with endonucleases WEN1 (2 × 10−8 M) and WEN2) (1.4 × 10−8) in the presence of 3 mM MgCl2 or in the absence of magnesium ions for 30 min or 2 h at 37°C. The hydrolysis products were separated by electrophoresis in 20% polyacrylamide gel in Tris-borate buffer containing 0.05 M EDTA. The gels were analyzed on a Fujifilm FLA 3000 Imager (Japan) at a wavelength of 413 nm.
To solution, containing 2 µg of unmethylated DNA λ phage (dcm−, dam−) and methylated by WAD methylase, 1 µL of buffer (corresponding for different restriction endonucleases) and 1 µL of commercial restriction endonucleases were added. The mixture was incubated at 37°C for 2 h. The hydrolysis products were analyzed in horizontal electrophoresis in 1.2% agarose.
Fractional centrifugation was used to isolate a fraction of closed-membrane vesicles and mitochondria from aging wheat coleoptiles. Electron microscopic examination of the fraction showed that it was a suspension of certain subcellular particles with a rather unusual organization [23]. These particles, surrounded by a membrane, were filled with a cytoplasmic matrix and contained one or more mitochondria (Figure 1).
Vesicles from coleoptiles were separated from nuclei and free mitochondria by fractional centrifugation in a sugar gradient. Because most vesicles contain more than one mitochondria, they sediment at lower g values than free mitochondria. This allowed us to isolate cytoplasmic structures that were not contaminated with free mitochondria.
Nuclease activity was extracted from cytoplasmic vesicles from coleptiles of 7-day-old etiolated wheat seedlings. Sequential chromatography using DEAE cellulose as well as Superdex-200, Superdex-75, and Toyopearl HW-50 columns led to the isolation of two protein fractions with nuclease activity [24],[25]. One of the enzymes, named WEN1, had an apparent molecular weight of about 27 kDa; the second enzyme, called WEN2, had an apparent molecular weight of about 43 kDa (Figure 2).
Plant endonucleases do not have a high substrate specificity; therefore, the products of DNA hydrolysis are presented in electrophoretograms in the form of smears. Based on analyses of the products of λ phage DNA hydrolysis, the WEN2 endonuclease prefers unmethylated λ phage DNA as a substrate. By contrast, WEN1 preferentially hydrolyzes methylated λ phage DNA (Figure 3).
Mg2+ and Ca2+ affect the activity of plant endonucleases. As shown in Figure 4, Mg2+ ions activate the action of both WEN1 and WEN2. In the presence of Mg2+ ions, an increase in the content of lower-molecular-weight products of DNA hydrolysis is observed. The composition of the products of DNA hydrolysis by endonucleases depends on the concentration of Mg2+ ions (data not shown). At a cation concentration of 1 mM in the reaction medium, oligonucleotide “smears” was seen in the gel; an increase in concentration to 3 mM was accompanied by a change in the composition of hydrolysis products, which mainly became oligonucleotides 120–140 bp in length. A further increase in the concentration of Mg2+ ions (above 10 mM) led to an increase in the proportion of higher-molecular-weight oligonucleotides, which indicates inhibition of enzyme activity. Thus, the optimal concentration of Mg2+ ions in the reaction medium was 3 mM. In the presence of Mg2+ ions, both endonucleases cleaved methylated and unmethylated DNA of λ phages almost equally with the formation of mainly oligonucleotides with a length of 120–140 bp. It can be assumed that magnesium ions cause endonucleases to lose sensitivity to DNA methylation status.
Ca2+ ions strongly activate WEN1 endonuclease, in a similar manner and almost to the same extent as Mg2+ ions in the hydrolysis of methylated DNA. However, it is interesting to note that, in the presence of bivalent cations, WEN1 endonuclease hydrolyzes unmethylated DNA almost into nucleotides. The composition of the hydrolysis products depends on the concentration of Ca2+ ions. An increase in the concentration above 5 mM is accompanied by inhibition of endonuclease WEN1 activity, which is expressed in the appearance of high-molecular-weight products (data not shown). Unlike WEN1, the activity of WEN2 is markedly inhibited by Ca2+ ions. Such a multidirectional dependence of WEN1 and WEN2 on Ca2+ ions (i.e., an increase in the concentration of Ca2+ ions) is optimal for one enzyme and acts as an inhibitor for the other, which is probably important for their functioning in the cell and affects the regulation of their activity.
The enzymatic methylation of DNA is carried out by specific cytosine and adenine DNA methyltransferases using SAM as a methyl group donor [31]. In the R-M system, the activity of some restriction endonucleases is modulated by the presence of SAM [32]. Due to the fact that endonucleases WEN1 and WEN2 are sensitive to the status of DNA methylation, we studied the possible effects of SAM on their activity (Figure 4C). We found that SAM inhibited the activity of WEN1 during the DNA hydrolysis reaction but significantly activated WEN2 regardless of methylation status, both during the hydrolysis of λ phage DNA (dam−, dcm−) and λ phage DNA (dam+, dcm+). In the presence of SAM, unmethylated DNA hydrolysis by WEN2 resulted in almost entirely oligonucleotides of 120–140 bp in size.
It can be assumed that there are specific regions responsible for binding to SAM in endonucleases WEN1 and WEN2, similar to restriction endonucleases. The different modulations of the activities of these endonucleases by allosteric effectors may indicate a different organization of enzymes, as well as their regulatory and possibly catalytic centers.
Thus, the DNA methylation status and the allosteric effects of SAM, a donor of methyl groups for methylation including DNA, are effective regulators of the nuclease activity of WEN1 and WEN2 in vitro, and possibly in vivo.
Nuclease activity was tested in mitochondria, nuclei, and in the vesicular fraction (Figure 4). Nuclease activity corresponding to the WEN1 endonuclease was found in all tested subcellular structures: free mitochondria, mitochondria in the vesicular fraction, and mitochondria in the nuclear fraction. Nuclease activity corresponding to WEN2 was found only in nuclear and vesicular fractions (Figure 4). In the process of seedling development, the nuclease activity corresponding to WEN2 increases in the nucleus until the fifth day of seedling growth (Figure 3). Then it begins to gradually accumulate in the vesicular fraction and reaches its maximum value on day 7 of seedling development (see Figure 4).
Nuclease activity corresponding to WEN1 is localized in free mitochondria in young seedlings. However, unlike WEN2, WEN1 is found in vesicles, which are already formed in coleoptiles on the third day of development of seedlings grown under etiolated conditions, albeit in insignificant amounts. With the further development of seedlings (day 5), the nuclease activity corresponding to WEN1 is already detected in the nuclear fraction. However, there are no exact data on the pathways of WEN1 transport into the nucleus: either part of the enzyme goes into the nucleus, and the rest into the vesicles, or the whole enzyme goes into the vesicles, and then some into the nucleus. However, the most realistic option is the first scenario.
Based on the dynamics of nuclease activity corresponding to WEN1 and WEN2, we developed a scheme of their localization in various subcellular structures during wheat seedling development (Figure 5). First, WEN1 in young seedlings is localized in free mitochondria. During the process of development, the enzyme is released from mitochondria and is transferred to the nuclear fraction and the vesicular fraction, which is a marker of apoptosis in plants. However, the appearance of WEN1 in the vesicular fraction together with mitochondria cannot be ruled out, because vesicles are formed through the formation of a dense membrane around mitochondria. WEN2, on the other hand, is localized in the nuclear fraction and moves to the vesicular fraction during seedling development (Figure 5).
Sequential purification of the extract from the vesicular fraction was carried out via ion-exchange chromatography using DEAE cellulose, gel filtration using Sephadex G-200 and G-50 columns, and chromatography using DNA cellulose resin. Elution was performed in 10 mM Tris-HCl buffer pH 7.0 in the presence of 5 mM EDTA. The use of 5 mM EDTA was needed to inactivate endonucleases. This made it possible to isolate the adenine DNA methyltransferase WAD in a homogeneous form; according to SDS electrophoresis, the apparent molecular weight of the enzyme was 39 kDa (Figure 2) [26].
Next, we analyzed the incorporation of a radioactive label from 3H-methyl-SAM into the adenine base of thymus DNA in wheat coleoptile extract, and found that its accumulation in coleoptiles reached a maximum value by day 7, after which it decreased (Figure 6).
The most probable localization of adenine DNA methyltransferase was found to be in the vesicular fraction (Figure 7).
The distribution of the r/a label between cytosine and adenine bases in subcellular structures (mitochondria, nuclei and vesicles) of 7 day-old wheat seedlings is shown in Table 1.
The label accumulated in the vesicular fraction of coleoptiles. It is interesting to note that the label accumulated to a much lower degree in the cytosine base in vesicles than in the adenine base. In addition, it mostly accumulated in young seedlings (3 days old) and in the DNA of the nuclear fraction. These results suggest that cytosine DNA methyltransferases exhibit nuclear localization, while adenine DNA methyltransferases are mainly in the mitochondria. In young wheat seedlings, adenine DNA methyltransferase is inactive or weakly active. During the development of the coleoptile with the formation of vesicles, some mitochondria pass into this fraction; during this period, the activity of adenine DNA methyltransferase increases.
Mitochondria | Nuclei | Vesicles | |
(Methyl)cytosine | 311 ± 12 | 2852 ± 48 | 183 ± 21 |
(Methyl)adenine | 392 ± 23 | 260 ± 19 | 962 ± 32 |
The DNA methyltransferase WAD methylates DNA in the presence of SAM to form N6-methyldeoxyadenosine, which was isolated by high-performance liquid chromatography in a gradient of 0–100% acetonitrile on С-18. The characteristic positions of the extrema of the UV absorption spectrum of the isolated base, corresponding to Vmax = 262 nm and Vmin = 236 nm, confirmed that N6-methyldeoxyadenosine formed during DNA methylation with the isolated enzyme, thus confirming that the enzyme was adenine DNA methyltransferase.
To determine the site specificity of WAD-methylase, we assessed the protective effect of methylated DNA of λ phage (dam−, dcm−) WAD-methylase against restriction by bacterial restriction endonucleases. This protective effect is manifested only against the restriction endonuclease BclI (Figure 8). This restriction endonuclease cleaves DNA at the 5′-TGATCA-3′ site; however, if the site is methylated at adenine, then the BclI endonuclease can no longer cleave it. Thorough analyses of the obtained λ phage DNA fragments after treatment with the MboI restriction endonuclease revealed the absence of low-molecular-weight DNA fragments compared to control samples. This slight difference is probably due to the fact that the GATC site is included in the TGATCA site, and after methylation, this site becomes inaccessible for cleavage by the MboI endonuclease. Comparative analyses of the restriction enzymes of unmethylated and methylated WAD methylase DNA obtained by hydrolysis with other restriction endonucleases did not reveal any differences in the composition and position of DNA fragments. Thus, the WAD methylase site 5′-TGATCA-3′ was determined.
Unlike in bacteria, endonucleases in plants do not have a pronounced site specificity of action. Although analyses of the products of λ phage DNA hydrolysis by endonucleases WEN1 and WEN2 did not reveal clear discrete bands (fragments) in agarose gel electrophoresis (Figure 3), the nature of the hydrolysis still indicated the existence of some specificity, for example, they did not cleave monotonic polymers and deoxyribooligonucleotides (oligos). WEN1 prefers to hydrolyze methylated DNA λ phage (dcm+, dam+), while WEN2 prefers unmethylated DNA λ phage (dcm−, dam−).
Unmethylated DNA λ phage (dcm−, dam−) was methylated at the adenine bases with the wheat DNA methyltransferase WAD at the 5′-TGATCF-3′ sites and at the cytosine bases with bacterial DNA methyltransferase SssI at the 5′-CpG-3′ sites. WEN1 hardly hydrolyzed DNA methylated at adenine bases (Figure 9); even an increase in the exposure time did not lead to new hydrolysis products. Hence, we concluded that WEN1 does not possess dam endonuclease specificity of action. At the same time, the enzyme hydrolyzed methylated DNA λ phage at cytosine bases even without cofactors (Figure 9).
In plants, the most common methylated site is CG [17]. Another methylated site in eukaryotic DNA is the CNG sequence; in plant DNA, more than 30% of 5-methylcytosine residues are localized precisely in these sequences [17]. 5-carboxyfluorescein (FAM) oligos have been synthesized in various contexts containing either a CG site or a CNG site. Versions containing methylcytosine at the CG and CNG sites have also been synthesized.
The endonuclease WEN1 weakly hydrolyzed FAM-oligos with the CG site, even with methylcytosine present. All FAM-oligos with CNG sites we used in any nearest surrounding nucleotide context, with the exception of the CCCG combination, were hydrolyzed by the WEN1 enzyme (Figure 9). Based on the results of analyses of the products of FAM oligo hydrolysis by the endonuclease WEN1, we concluded that the enzyme prefers to hydrolyze oligos with a CNG site and with a methylated cytosine base inside the site between the meC and N bases.
The endonuclease WEN2 differs in specificity from WEN1. WEN2 hydrolyzes FAM-oligos with CG sequences only if the oligo contains a GAT site. The enzyme cleaved the oligo in front of the GAT site regardless of context (Figure 9). However, hydrolysis did not occur if the oligo was methylated at the adenine base. Thus, WEN2 preferentially cleaves CG sequences directly adjacent to the unmethylated recognition site, similar to type III restriction endonucleases [1].
The coleoptile is the first germinal (colorless, green or reddish) leaf of cereals that does not have a leaf blade and is a closed tube. It has protective properties for subsequent leaves, functions for a relatively short period of time, and quickly dies as the seedling forms and grows. In vacuoles of coleoptile cells of wheat seedlings, numerous cytoplasmic regions of various size surrounded by a closed membrane and containing one or several mitochondria, fragments of membranes of the rough endoplasmic reticulum, and polyribosomal complexes have been found [23]. These are isolated cytoplasmic structures not associated with the main cytoplasm of the cell. The cytoplasm in the detected vesicles has no signs of destruction, in contrast to the contents of the surrounding vacuole [23].
WEN1 and WEN2 are eukaryotic endonucleases that recognize substrate DNA according to their methylation status. This phenomenon is common for highly specific bacterial and protozoal (Chlamidomonas) restriction endonucleases belonging to the R-M system [4],[33]. During the DNA hydrolysis reaction, SAM inhibits the activity of WEN1 regardless of methylation status but significantly activates WEN2, both during the hydrolysis of λ phage DNA (dam−, dcm−) and λ phage DNA (dam+, dcm+). In the presence of SAM, unmethylated DNA hydrolysis by WEN2 results in almost entirely oligonucleotides 120–140 bp in size.
It can be assumed that there are specific regions responsible for binding to SAM in the WEN1 and WEN2 endonucleases, similar to restriction endonucleases. The different modulations of their activities by allosteric effectors may indicate a different organization of enzymes, as well as their regulatory and possibly catalytic centers.
Ions of divalent metals are essential components involved in the catalytic hydrolysis of substrates in most nucleases. Mg2+ and Ca2+ are the most common divalent cations in living organisms [34],[35], and Mg2+ ions are predominantly localized inside the cell [36]. Other ions such as Fe2+, Zn2+, and Cu2+ are also widespread and actively involved in cellular processes. The ions Mn2+ and Ni2+ are also essential for the functioning of organisms, but in cells, they are found in lower concentrations than Mg2+ and Ca2+ [35].
WEN1 and WEN2 are neutral Ca2+, Mg2+-dependent enzymes. Mg2+ ions activate both of them. In the presence of Mg2+ ions, an increase in the yield of lower-molecular-weight products of DNA hydrolysis is observed. Ca2+ ions strongly activate WEN1 in the same way and almost to the same extent as Mg2+ ions. However, they markedly inhibit WEN2. This multidirectional dependence of the action of WEN1 and WEN2 on Ca2+ ions is probably important for their function in the cell and is aimed at regulating their activity: an increase in the concentration of Ca2 + ions is optimal for one enzyme and inhibits the other.
Thus, the status of DNA methylation and the allosteric effects of SAM, a donor of methyl groups for methylation including DNA, are effective regulators of the nuclease activity of WEN1 and WEN2 in vitro, and possibly in vivo.
The bacterial R-M system includes a highly specific restriction endonuclease and DNA methyltransferase [1],[2]. We do not know exactly what the role of the R-M system in plants might be. However, we speculate that, because pathogens can trigger programmed cell death (PCD) in plants and because a dying cell is an excellent target for attack by pathogens, vesicles are formed to protect the mitochondrial genome from the penetration of foreign DNA. One of the mechanisms of protection against foreign DNA in bacteria is the process of DNA methylation and restriction. In wheat seedlings, the vesicular fraction contains DNA methyltransferase, which methylates adenine at the TGm6ATCA site, and the WEN2 endonuclease, which has a GAT recognition site and hydrolyzes the CG (GC) bond in front of the recognition site only if it is not methylated. Thus, it can be assumed that these two enzymes are interconnected and may form an R-M system analogous to that observed in bacteria, or the individual elements of such a system. We assigned the R-M system in wheat to type IV, because the WEN2 endonuclease does not have such a high specificity as the restriction endonucleases, and the WEN2 recognition site (GAT) is only a part of the WAD methylase methylation site (TGATCA).
We do not know exactly what may be the role of the R-M system in plants. However, accepting the fact that pathogens can launch PCD in plants, and that a dying cell is an excellent target for attack by pathogens, it can be imagined that vesicles are formed to protect the mitochondrial genome from the penetration of foreign DNA. One of the defense mechanisms against foreign DNA in bacteria is the process of DNA methylation and restriction. The bacterial R-M system includes a highly specific restriction endonuclease and DNA methyltransferase [1],[2]. In wheat seedlings, DNA methyltransferase is found in the vesicular fraction and methylates adenine at the TGm6ATCA site, where WEN2, which has a GAT recognition site, hydrolyzes the CG (GC) bond in front of the recognition site only if it is not methylated. Therefore, these two enzymes can be considered to be interconnected and to possibly serve as an R-M system. Thus, it can be considered that these two enzymes are related to each other and they can be attributed to an R-M system similar to bacterial. We assigned the R-M system in wheat to type IV, because the WEN2 endonuclease does not have such a high specificity as the restriction endonucleases, and the WEN2 recognition site (GAT) is only a part of the WAD methylase methylation site (TGATCA). Strong evidence of the protective role of this system in plants is the active process of replication in mitochondria localized to vesicles, while the fragmentation of nuclear material actively occurs in the cell [37]. Adenine-DNA methyltransferase is involved in the replication process, and wheat mitochondrial DNA contains about 1% N6-A of all adenine bases [38],[39].
The formation of vesicles is necessary for the cell to preserve the genetic material located in the mitochondria. The presence of two endonucleases that are sensitive to the status of DNA methylation, namely, WEN1 and WEN2, and adenine DNA methyltransferase in the vesicular fraction is most likely associated with the existence of the epigenetic control (DNA methylation) of nuclease activity in plants. Multidirectional sensitivity to the status of DNA methylation and to the presence of cofactors SAMa and Ca2+ ions suggests the participation of WEN1 and WEN2 in mitochondrial DNA protection. WEN2 has a GAT recognition site, which is part of the methylation site of adenine DNA, and it hydrolyzes the CG (GC) bond upstream of the recognition site only if it is not methylated. Based on the relationship between the two enzymes, we assume that an R-M system similar to that of the type IV observed in bacteria, or some of its elements, may exist in plants. However, this system appears in cells formed at an early stage of PCD in an unusual vesicular fraction. It can be assumed that this system in plants is part of the immune response to the action of a pathogen and is aimed at protecting the cellular material.
[1] |
Rao DN, Dryden DTF, Bheemanaik S (2014) Type III restriction-modification enzymes: a historical perspective. Nucleic Acids Res 42: 45-55. doi: 10.1093/nar/gkt616
![]() |
[2] |
Loenen WAM, Raleigh EA (2014) The other face of restriction: modification-dependent enzymes. Nucleic Acids Res 42: 56-69. doi: 10.1093/nar/gkt747
![]() |
[3] |
Dixon M, Fauman EB, Ludwig ML (1999) The black sheep of the family: AdoMet-dependent methyltransferases that do not fit the consensus structural fold. S-Adenosylmethionine-dependent Methyltransferases: Structures and Functions World Scientific Inc. Singapore: 39-54. doi: 10.1142/9789812813077_0002
![]() |
[4] |
Wilson GG, Murray NE (1991) Restriction and modification systems. Annu Rev Genet 5: 585-627. doi: 10.1146/annurev.ge.25.120191.003101
![]() |
[5] |
Arber W, Dussoix D (1962) Host specificity of DNA produced by Escherichia coli. I. Host controlled modification of bacteriophage lambda. J Mol Biol 5: 18-36. doi: 10.1016/S0022-2836(62)80058-8
![]() |
[6] |
Roberts RJ, Belfort M, Bestor T, et al. (2003) A nomenclature for restriction enzymes, DNA methyltransferases, homing endonucleases and their genes. Nucleic Acids Res 31: 1805-1812. doi: 10.1093/nar/gkg274
![]() |
[7] | Marinus MGMethylation of DNA. Escherichia coli and Salmonella typhimurium (1996) .Washington DC: ASM Press, 782-791. |
[8] |
Peterson KR, Wertman KF, Mount DW, et al. (1985) Viability of Escherichia coli K-12 DNA adenine methylase (dam) mutants requires increased expression of specific genes in the SOS regulon. Mol Gen Gene 201: 14-19. doi: 10.1007/BF00397979
![]() |
[9] |
Lieb M, Bhagwat AS (1996) Very short patch repair: Reducing the cost of cytosine methylation. Mol Microbiol 20: 467-473. doi: 10.1046/j.1365-2958.1996.5291066.x
![]() |
[10] |
Ban C, Yang W (1998) Structural basis for MutH activation in E. coli mismatch repair and relationship of MutH to restriction endonucleases. EMBO J 17: 1526-1534. doi: 10.1093/emboj/17.5.1526
![]() |
[11] |
Ahmad I, Rao DN (1996) Chemistry and biology of DNA methyltransferases. Crit Rev Biochem Mol Biol 31: 361-380. doi: 10.3109/10409239609108722
![]() |
[12] |
Gong W, O'Gara M, Blumenthal RM, et al. (1997) Structure of PvuII DNA-(cytosine N4) methyltransferase, an example of domain permutation and protein fold assignment. Nucleic Acids Res 25: 2702-2715. doi: 10.1093/nar/25.14.2702
![]() |
[13] |
Stoddard BL (2005) Homing endonuclease structure and function. Q Rev Biophys 38: 49-95. doi: 10.1017/S0033583505004063
![]() |
[14] |
Spiegel PC, Chevalier B, Sussman D, et al. (2006) The structure of I-CeuI homing endonuclease: Evolving asymmetric DNA recognition from a symmetric protein scaffold. Structure 14: 869-880. doi: 10.1016/j.str.2006.03.009
![]() |
[15] | Blow MJ, Clark TA, Daum CG, et al. (2016) The Epigenomic Landscape of Prokaryotes. PLoS Genet 12. |
[16] |
Vasu K, Nagaraja V (2013) Diverse functions of restriction-modification systems in addition to cellular defense. Microbiol Mol Biol Rev 77: 53-72. doi: 10.1128/MMBR.00044-12
![]() |
[17] | Vanyushin BF, Ashapkin VV (2009) DNA Methylation in Plants New York: Nova Science Publishers Inc. |
[18] | Vanyushin BF, Ashapkin VV (2007) Peculiarities of DNA methylation in plants. Progress in DNA Methylation Research New York: Nova Science Publishers Inc., 1-90. |
[19] |
Goll MG, Bestor TH (2005) Eukaryotic cytosine methyltransferases. Annu Rev Biochem 74: 481-514. doi: 10.1146/annurev.biochem.74.010904.153721
![]() |
[20] |
Lister R, Pelizzola M, Dowen RH, et al. (2009) Human DNA methylomes at base resolution show widespread epigenomic differences. Nature 462: 315-322. doi: 10.1038/nature08514
![]() |
[21] |
Lister R, Mukamel EA, Nery JR, et al. (2013) Global epigenomic reconfiguration during mammalian brain development. Science 341: 1237905. doi: 10.1126/science.1237905
![]() |
[22] |
Greer EL, Blanco MA, Gu L, et al. (2015) DNA Methylation on N6-Adenine in C. elegans. Cell 7: 868-878. doi: 10.1016/j.cell.2015.04.005
![]() |
[23] |
Bakeeva LE, Kirnos MD, Aleksandrushkina NI, et al. (1999) Subcellular reorganization of mitochondria producing heavy DNA in aging wheat coleoptiles. FEBS Lett 457: 122-125. doi: 10.1016/S0014-5793(99)01025-X
![]() |
[24] |
Fedoreyeva LI, Sobolev DE, Vanyushin BF (2007) Wheat endonuclease WEN1 depent on S-adenosyl-L-methionine and sensitive to DNA methylation status. Epigenetics 2: 50-53. doi: 10.4161/epi.2.1.3933
![]() |
[25] | Fedoreyeva LI, Sobolev DE, Vanyushin BF (2008) S-adenosyl-L-methionine dependent and sensitive to the status of DNA methylation endonuclease WEN2 from wheat coleoptiles. Biochemistry (Russian) 73: 1243-1251. |
[26] |
Fedoreyeva LI, Vanyushin B F (2002) N6-Adenine DNA-methyltransferase in wheat seedlings. FEBS Lett 514: 305-308. doi: 10.1016/S0014-5793(02)02384-0
![]() |
[27] | Kirnos MD, Aleksandrushkina NI, Vanyushin BF (1997) Apoptosis in cells of the first leaf and coleoptile of wheat seedlings: internucleosomal fragmentation of the genome and synthesis of heavy oligonucleosomal size of DNA fragments. Biochemistry (Russian) 62: 1008-1014. |
[28] |
Bradford MM (1976) A rapid and sensitive method for the quantitation of microgram quantities of protein utilizing the principle of protein-dye binding. Analyt Biochem 72: 248-254. doi: 10.1016/0003-2697(76)90527-3
![]() |
[29] |
Laemmli UK (1970) Cleavage of structural proteins during the assembly of the head of bacteriophage T4. Nature 227: 680-685. doi: 10.1038/227680a0
![]() |
[30] |
Bannister D, Glover SW (1968) Restriction and modification of bacteriophages by R strains of Escherichia coli K-12. Biochem Biophys Res Commun 30: 735-738. doi: 10.1016/0006-291X(68)90575-5
![]() |
[31] |
Vertino PM (1999) Eukaryotic DNA methyltransferases. S-Adenosylmethionine-dependent Methyltransferases: Structures and Functions 341-372. doi: 10.1142/9789812813077_0012
![]() |
[32] |
Bist P, Sistla S, Krishnamurthy V, et al. (2001) S-Adenosyl-L-methionine is required for DNA cleavage by type III restriction enzymes. J Mol Biol 310: 93-109. doi: 10.1006/jmbi.2001.4744
![]() |
[33] |
Cowan JA (2002) Structural and catalytic chemistry of magnesium-dependent enzymes. Biometals 15: 225-235. doi: 10.1023/A:1016022730880
![]() |
[34] | Lyons TJ, Eide DJ (2006) Transport and storage of metal ions in biology. Biological Inorganic Chemistry: Structure and Reactivity 57-77. |
[35] |
Maguire ME, Cowan JA (2002) Magnesium chemistry and biochemistry. Biometals 15: 203-210. doi: 10.1023/A:1016058229972
![]() |
[36] |
Romani A, Scarpa A (1992) Regulation of cell magnesium. Arch Biochem Biophys 298: 1-12. doi: 10.1016/0003-9861(92)90086-C
![]() |
[37] | Kirnos MD, Aleksandrushkina NI, Goremykin VV, et al. (1992) “Heavy” mitochondrial DNA in higher plants. Biochemistry (Russian) 57: 1566-1673. |
[38] | Aleksandrushkina NI, Kudryashova IB, Kirnos MD, et al. (1990) Synthesis and methylation at adenine residues of “heavy” miniplasmids of mitochondrial DNA in coleoptile and leaves of wheat seedlings. The influence of phytohormones. Biochemistry (Russian) 55: 2038-2045. |
[39] |
Kirnos MD, Alexandrushkina NI, Zagorskaya GYa, et al. (1992) Superproduction of heavy minicircular mitochondrial DNA in aging wheat coleoptiles. FEBS Lett 298: 109-112. doi: 10.1016/0014-5793(92)80033-D
![]() |
1. | Irma A. Jiménez-Ramírez, Gema Pijeira-Fernández, Delia M. Moreno-Cálix, Clelia De-la-Peña, Same modification, different location: the mythical role of N6-adenine methylation in plant genomes, 2022, 256, 0032-0935, 10.1007/s00425-022-03926-y |
Mitochondria | Nuclei | Vesicles | |
(Methyl)cytosine | 311 ± 12 | 2852 ± 48 | 183 ± 21 |
(Methyl)adenine | 392 ± 23 | 260 ± 19 | 962 ± 32 |