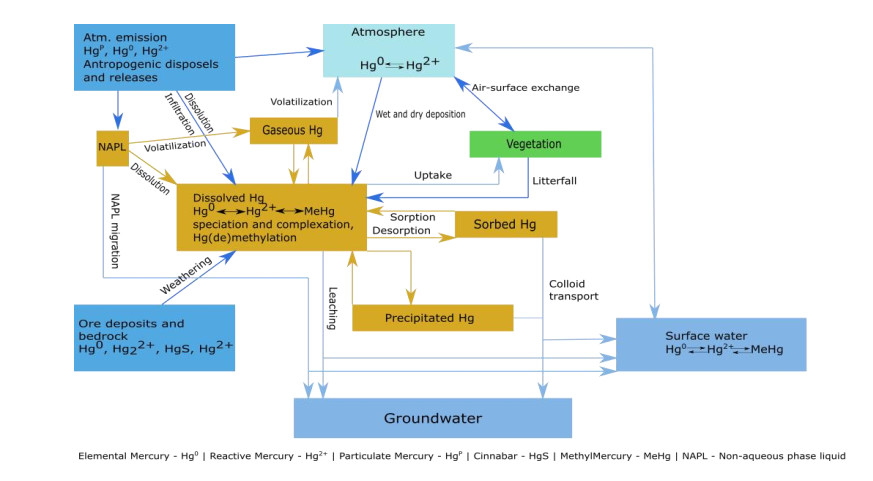
Our paper reviews the current understanding of mercury in the environment of soil and sediment, including sampling, mobilization phases and analyzing methods. As a dangerous trace element, mercury has been shown to have several harmful effects on the environment. Mercury is released into the environment in a variety of chemical forms by both geogenic and human activities, with the majority of it coming from anthropogenic sources. It is affected by environmental conditions such as pH, redox potential, light and temperature-all of which determine its final chemical form-reactivity and toxicity. Methylmercury is considered one of the most poisonous forms found in nature. Considering the methodologies of the studies carried out we have found that the best technique for preserving methylmercury in soil and sediment samples is to freeze it immediately after collection. Organically rich soils are related to higher total mercury levels. Plants, such as Solanum nigrum (BR3) and Cynodon dactylon (BR2), can play an important role in mercury transport and accumulation. Solid-phase selenium causes faster demethylation and slower methylation of mercury. Methylmercury can increase by climate change and thawing; arctic permafrost is a potential source of Hg. Chemical vapor generation inductively coupled plasma mass spectrometry was used to develop a simple and quick method for measuring methylmercury; ultrasonic agitation and HNO3 were used for the process, the last of which proved to be the most efficient for selective extraction of methylmercury.
Citation: Gytautas Ignatavičius, Murat H. Unsal, Peter E. Busher, Stanisław Wołkowicz, Jonas Satkūnas, Giedrė Šulijienė, Vaidotas Valskys. Geochemistry of mercury in soils and water sediments[J]. AIMS Environmental Science, 2022, 9(3): 277-297. doi: 10.3934/environsci.2022019
[1] | Martina Grifoni, Francesca Pedron, Gianniantonio Petruzzelli, Irene Rosellini, Meri Barbafieri, Elisabetta Franchi, Roberto Bagatin . Assessment of repeated harvests on mercury and arsenic phytoextraction in a multi-contaminated industrial soil. AIMS Environmental Science, 2017, 4(2): 187-205. doi: 10.3934/environsci.2017.2.187 |
[2] | Theresa Vertigan, Kriya Dunlap, Arleigh Reynolds, Lawrence Duffy . Effects of Methylmercury exposure in 3T3-L1 Adipocytes. AIMS Environmental Science, 2017, 4(1): 94-111. doi: 10.3934/environsci.2017.1.94 |
[3] | Romilda Z. Boerleider, Nel Roeleveld, Paul T.J. Scheepers . Human biological monitoring of mercury for exposure assessment. AIMS Environmental Science, 2017, 4(2): 251-276. doi: 10.3934/environsci.2017.2.251 |
[4] | Cristina Calderón-Tapia, Edinson Medina-Barrera, Nelson Chuquin-Vasco, Jorge Vasco-Vasco, Juan Chuquin-Vasco, Sebastian Guerrero-Luzuriaga . Exploration of bacterial strains with bioremediation potential for mercury and cyanide from mine tailings in "San Carlos de las Minas, Ecuador". AIMS Environmental Science, 2024, 11(3): 381-400. doi: 10.3934/environsci.2024019 |
[5] | Lei Wang, Huan Du, Jiajun Wu, Wei Gao, Linna Suo, Dan Wei, Liang Jin, Jianli Ding, Jianzhi Xie, Zhizhuang An . Characteristics of soil erosion in different land-use patterns under natural rainfall. AIMS Environmental Science, 2022, 9(3): 309-324. doi: 10.3934/environsci.2022021 |
[6] | Sandrine Chifflet, Marc Tedetti, Hana Zouch, Rania Fourati, Hatem Zaghden, Boubaker Elleuch, Marianne Quéméneur, Fatma Karray, Sami Sayadi . Dynamics of trace metals in a shallow coastal ecosystem: insights from the Gulf of Gabès (southern Mediterranean Sea). AIMS Environmental Science, 2019, 6(4): 277-297. doi: 10.3934/environsci.2019.4.277 |
[7] | Jerry R. Miller, John P. Gannon, Kyle Corcoran . Concentrations, mobility, and potential ecological risks of selected metals within compost amended, reclaimed coal mine soils, tropical South Sumatra, Indonesia. AIMS Environmental Science, 2019, 6(4): 298-325. doi: 10.3934/environsci.2019.4.298 |
[8] | Francesco Teodori . Health physics calculation framework for environmental impact assessment of radiological contamination. AIMS Environmental Science, 2021, 8(4): 403-420. doi: 10.3934/environsci.2021026 |
[9] | Joseph A. Kazery, Dayakar P. Nittala, H. Anwar Ahmad . Meteorological effects on trace element solubility in Mississippi coastal wetlands. AIMS Environmental Science, 2019, 6(1): 1-13. doi: 10.3934/environsci.2019.1.1 |
[10] | Oltion Marko, Joana Gjipalaj, Dritan Profka, Neritan Shkodrani . Soil erosion estimation using Erosion Potential Method in the Vjosa River Basin, Albania. AIMS Environmental Science, 2023, 10(1): 191-205. doi: 10.3934/environsci.2023011 |
Our paper reviews the current understanding of mercury in the environment of soil and sediment, including sampling, mobilization phases and analyzing methods. As a dangerous trace element, mercury has been shown to have several harmful effects on the environment. Mercury is released into the environment in a variety of chemical forms by both geogenic and human activities, with the majority of it coming from anthropogenic sources. It is affected by environmental conditions such as pH, redox potential, light and temperature-all of which determine its final chemical form-reactivity and toxicity. Methylmercury is considered one of the most poisonous forms found in nature. Considering the methodologies of the studies carried out we have found that the best technique for preserving methylmercury in soil and sediment samples is to freeze it immediately after collection. Organically rich soils are related to higher total mercury levels. Plants, such as Solanum nigrum (BR3) and Cynodon dactylon (BR2), can play an important role in mercury transport and accumulation. Solid-phase selenium causes faster demethylation and slower methylation of mercury. Methylmercury can increase by climate change and thawing; arctic permafrost is a potential source of Hg. Chemical vapor generation inductively coupled plasma mass spectrometry was used to develop a simple and quick method for measuring methylmercury; ultrasonic agitation and HNO3 were used for the process, the last of which proved to be the most efficient for selective extraction of methylmercury.
Sediment is a vital and dynamic part of river basins, estuaries, and seas like the Baltic Sea; it is a semi-closed, inland water basin that is extremely vulnerable to negative environmental pressures from the surrounding populated and industrialized areas [1]. Water exchange with the Atlantic Ocean is low and mostly occurs as a result of inflows from the North Sea via the Danish Straits [2,3]. Thus, mercury entering the Baltic Sea basin stays in the Baltic Sea basin. It is formed of several shallow bays and estuaries, including the Gulf of Finland, the Gulf of Riga, the Bay of Gdansk, the Sea of Bothnia, and the Bay of Bothnia, with a sand and clay mineral-rich bottom. This sea formed 130, 000 years ago and has evolved through numerous stages, including an ice age lasting around 100, 000 years during the Weichselian glacial period [4]. In the Baltic Sea basin, sediments are transported to the sea via rivers, and over 70% of the mercury that enters the Baltic Sea basin comes via rivers, while only 30% enters from sources of wet and dry air deposition and shoreline erosion [2,3,5]. Because of this mercury-contaminated sediments entering the Baltic Sea may be from either the immediate coastal area or from sources of mercury pollution that exist along the rivers. As a result of riverine transport, mercury released from polluted sites into the hydrosphere related to leaching and erosion eventually reaches the Baltic Sea [6]. Due to a lack of sufficient data, the quantity of mercury that enters aquatic habitats because of erosion and riverine transport is uncertain and difficult to establish [7]. This accumulated, but often unaccounted for, mercury-contaminated sediment load may have acutely harmful effects on the environment, living organisms and the economy [8].
While heavy metals in aquatic ecosystems that ultimately accumulate in sediments may come from both natural and anthropogenic sources, most are due to human activities [9]. Point sources of the Baltic Sea, such as coal and metal ore mines (in Germany and Poland), steel and metal industry (in Estonia, Germany, Poland, Russia, and Sweden), wood and paper mills (in Finland and Sweden), and ammunition and military waste dumpsites (in the Bornholm, Gotland, Gdansk, Kolberg Heide, Mecklenburg Bay, and Adlergrud) and wrecks of ships and planes [2,3] all contribute to the heavy metal deposition in Baltic Sea sediments. In the Gulf of Gdansk and the Bay of Puck in Poland, atmospheric deposition, river discharge, shipyards, harbors, wastewater treatment plants, and the municipal areas of Gdansk, Gdynia, and Sopot were found to be the primary sources of mercury [10]. Additionally, high mercury concentrations have been found in the Baltic Sea basin near industrial and military waste disposal sites [11]. The Baltic Sea's general environmental health has declined significantly since the 1950s, when new businesses were established on its coastlines following World War II, particularly on the Baltic Sea's East and south coasts. The huge industrial load on the coastal estuaries has caused a catastrophic ecosystem degradation in the Sea of Bothnia, resulting in the largest accumulation of pollutants dumped on the shores of any Scandinavian country in history [4].
While atmospheric mercury deposition into the Baltic Sea has declined since the 1990s [12], the remobilization of mercury from the bottom sediments has increased [13]. Floods and other severe weather events (potentially related to anthropogenic climate change) continue to contribute to the increased mercury remobilization by leaching sub-level deposits [5]. Frequent storms and increasing sea levels have a significant effect on influencing the forming of the shorelines [13].
In marine systems, sediments are the primary sink for mercury contamination [3,14,15]. Mercury concentration in bottom sediments is a good indication of contamination in water. It accumulates in bottom sediments because of simple sedimentation; however, it is freed from the sediments and becomes accessible for further biogeochemical transformations [16]. Several physicochemical processes influence the form of mercury in marine sediments, including adsorption onto clay minerals and organic matter, the formation of complexes with organic and inorganic ligands, precipitation and coprecipitation (primarily mercury sulfide), oxidation and reduction reactions, and the bacterial mediated formation of the most dangerous metal-organic compounds (mainly methylmercury) [2]. Baltic Sea's geology has an important effect on pollutants, as clay materials found in the Baltic are very similar to clays used for decontamination and remediation in polluted waters and soil because of their high sorption feature [17,18]. The geophysical qualities of the Baltic Sea facilitate the absorption of pollutants of both organic and inorganic origin, and it can operate as an accumulator of water-soluble and insoluble pollutants emitted by industrial sites along the shorelines [19]. In uncontaminated sediments, total mercury (THg) concentration is between 0.2 to 0.4 mg∙kg-1, however, in sediments close to industrial areas and cities, THg concentration can be up to 100 mg∙kg-1 and 0.1 mg∙kg-1 methylmercury [20].
Soil is a dynamic medium created by a porous matrix in which air, water, and biota intermix. Alterations in soil processes contribute to changes in ecosystem functioning and many environmental problems arise from the dynamic balance between inorganic and organic substances in the soil [21]. To maintain quality and functions all soil types must exist in a sustainable state that is negatively influenced by the presence of heavy metals such as mercury [22].
Mercury is a hazard to the natural environment and human health because of its toxicity, persistence and ability to bioaccumulate, so they do not degrade and have a tendency to bioaccumulate. The natural concentration and dynamics of soil, as well as human activities, have been reported as influencing the presence of these components in greenhouse soils (GS). Mercury is not abundant in nature, with a mean concentration of 0.05 mg kg-1 in the Earth's crust [23]. Mercury presented a high correlation with agriculture in a 2008 study and the use of fertilizer, manure, and agrochemicals in European soil are significant mercury sources [24]. Long et al. [25] investigated the spatial-temporal changes of heavy metals in Shanghai farming soils and discovered that mercury contributed to pollution. Ramos-Miras et al. [23] investigated the behavior of GS and grouped samples according to age. The result for mercury was 0.0371 mg∙kg-1, and 74% of GS surpassed the background level and the reason was the accumulation of mercury as a result of farming techniques used in the last 35 years. Conversely, a recent study shows that mercury in the soil is closely associated mainly with natural processes (high soil organic carbon, vegetation, temperature, soil texture, and pH) and obvious high values at historical mining activity and coal combustion sites [26,27]. According to Martin et al. [28] coal-fired power station of Alcudia in Majorca, mercury content in the topsoil has doubled in 11 years. On the whole island, The Alcudia coal-fired power station had a higher concentration (above 100 g∙kg-1). The majority of the mercury released was deposited within 15 kilometers of the source. The THg stock in Majorcan soil rose from 432.96 tons to 493.18 tons over 11 years. The impacts of mercury emissions from coal-burning power plants are becoming more noticeable every year and it can be a serious problem in the future if the trend continues and according to Medynska-Juraszek and Kabala [27], another reason is for mercury contamination in Poland's soils is mining activities. Also, Ballabio et al. [26] mentioned that in Europe, there were 209 hotspots of mercury with values greater than 0.422 mg∙kg-1, with mining responsible for 42% of them.
Soils act as mercury sinks similar to aquatic sediments, and also are sources of atmospheric mercury. For this reason, soils play an important role in global mercury cycling. The European Monitoring and Evaluation Program (EMEP) provides data on annual averages of mercury concentrations in air and annual averages of mercury depositions [29]. The bioavailability and toxicity of heavy metals are connected to the total concentration in soils as well as numerous other environmental factors [24]. Soils are so vital to ecosystem function that when soils are degraded by pollution or other factors, the rest of the entire ecological complex suffers and may collapse [30]. The quantity of mercury mass accumulating in soils throughout the world is enormous, estimated to be in the range of 250–1000 Gigagram (Gg). Even though mercury is naturally found in soils from geologic sources or through natural events such as forest fires and volcanic eruptions, anthropogenic influences are responsible for the majority of that mercury, with an estimated 86 Gg of anthropogenic mercury emissions now accumulated in surface soils [31]. The mercury pool in Europe's topsoil is predicted to be 44.8 Gg [26].
One example from Europe, Poland is one of Europe's major mercury emitters, and THg concentrations in its abiotic environment remain high [32]. Every year Poland releases an estimated 40 tons of mercury into the atmosphere. In Poland, mercury pollution of soils is linked to hot spots, which are frequently found near chemical factories (chloralkaline plants, coal power plants, metallurgic plants), where hard coal and lignite combustion processes provide energy (these account for 44% and 18.3% of mercury pollution respectively). Other sources, such as the production of cement and disposal of damaged fluorescent bulbs, are responsible for 16.6% and 6.4% of the THg released in Poland [33,34]. Poland is attempting to reduce the impact of these activities and has recently implemented numerous regulations that have significantly reduced mercury emissions to the environment [32]. In 1995, THg emissions from anthropogenic sources such as coal combustion and in total were calculated to be 31.90 and 33.60 tons, respectively and for Lithuania, it was only 0.1 tons in sum [35]. Whereas THg emissions in 2005, in Poland, was 20.1 tons and in Lithuania was 0.37 tons, in total [36,37]. Moreover, when compared 2005 to 2012 emissions, 26% more mercury was released into the atmosphere [38]. During the period 2007–2017, the mercury load was higher in the west of Lithuania (Žemaitija) than in the east of Lithuania (Akštaitija) [39]. Although 1.3 times larger than Slovakia and almost 3 times larger than Slovenia, Lithuania's mercury stock is less due to its mercury density [7].
Due to anthropogenic and natural activities over time mercury accumulates in soils, ocean sediments and in lake and river sediments [34]. The major negative environmental impact of mercury accumulation is that it may be converted into methylmercury, which is a highly toxic form of mercury [40]. It should not be forgotten that climate change also influences mercury. European Environmental Agency (EEA) [41] has indicated that climate change will raise the risk of mercury. Also, Schuster et al. [42] mentioned that permafrost (frozen soil) is also an important source of mercury emissions and permafrost retains significant quantities of mercury, which could be released in the future.
In this study, we summarize our current knowledge and methods associated with understanding mercury in the environment.
General considerations: Accurate and continuous environmental monitoring for mercury is critical to protecting human and environmental health, which are intimately and directly interconnected [43]. Environmental monitoring has become more important in recent years due to mounting pressure on ecological systems primarily caused by the increasing human population, increasing urbanization and increasing industrialization. Environmental monitoring is very broad and needs a multidisciplinary scientific strategy [44].
Why monitor soils: Environmental monitoring of soils involves the systematic determination of the inorganic and organic components in specific soil types to document both composition and temporal changes. This includes documentation of the presence and concentration levels of pollutants. Early detection of changes and the impact they have on soil quality is vital to assure soil viability. Soil monitoring allows policy initiatives to be designed and implemented assuring the preservation of soils [45,46]. It is necessary to monitor variations in the range of the different soil types present in a region, to enable the development of evidence-based policies that encourage sustainable soil management. Furthermore, in addition to national soil monitoring networks (SMN), an EU-wide monitoring effort is conducted by the statistical office of the European Union [47].
Why monitor sediments: Sediment monitoring provides a baseline of general sediment composition and documents any long-term changes due to anthropogenic impacts [48]. Also, contaminant toxicity, bioavailability, and bioaccumulation that occur when pollutants migrate between sediments and water are important aspects of sediment monitoring [49]. The European sediments network (SedNet) is designed to incorporate sediments issues and expertise into European strategies to encourage the achievement of good environmental performance and to create new sediments management tools [50].
Summary of sampling methods: Since sampling attempts to reflect the analyte(s), it is possible to determine the amount of concentration or the existence of an undesirable contaminant. Physically collecting a sample, contains a sampling protocol with specific steps [51].
International Atomic Energy Agency (IAEA) [52,53] and Artiola et al. [44], describe numerous, specific sampling techniques, two-stage, cluster, judgmental, random, stratified random, systematic grid, search and transect are some of the approaches for the sampling and should be consulted when designing a specific sampling protocol. Briefly, two-stage sampling entails dividing an area into regular components. Grid sampling involves taking samples at regular intervals and at defined spacings. Simple random sampling is the collecting of samples at an arbitrary. Judgmental sampling is the process of selecting sample locations at a site based on historical data and visual examination. Stratified random sampling is dividing the sample region into smaller portions known as strata, based on historical data and preceding analytical results. Search sampling is performed for areas where pollutants exceed the applicable criteria of the hot spots. It is utilized either systematic grid or systematic random sampling. Transect sampling involves creating one or more transect lines across a site's surface.
Collection and pre-treatment of samples: To limit sample contamination/degradation or undesired chemical reactions during the collection, pre-treatment, and storage phases of environmental sampling, tools and containers made of polymers, glass, stainless steel, or aluminum are required. Sampling implements and containers made of polytetrafluoroethylene (PTFE), fluorinated polyethylene (FLPE), polyethylene terephthalate (PET), Pyrex glass, and quartz containers are recommended for the collection and storage of mercury solutions. PET is inexpensive, recyclable, lightweight, and does not break down easily [54], while PTFE vessels will reduce the loss of analytes due to adsorption on the surface of the containers [55]. Due to the rapid degradation of organic mercury, species interconversion, and surface adsorption, polyethylene (PE) containers are not recommended.
Storage of samples: In order to protect the analytes, the samples should be pre-treated with an acidic solution, which avoids the interconversion of the mercury species. A thin layer of water can also be used to cover sediments from the site and seal with no headspace. Soil samples were mostly packed in ice-containing cooler PE bags, and transferred to the laboratory, dried at lower than 60 ℃, crushed, homogenized, sieved, and stored in PE containers at 4 ℃. Since drying conditions may have an impact on the mercury content of soil samples, freeze-drying is preferred over air and oven drying [54,56]. However, in research comparing mercury concentrations in moist vs. dry samples (dried at 40 ℃) Gilli et al. [57] found little impact of drying on concentration values.
For relevant analytical results, proper sample storage and preparation methods are critical. Because soils and sediments contain water at different levels and are inhomogeneous, sample preparation processes such as drying, and grinding are required before analysis [58]. Since drying conditions have a large impact on the mercury content of soil samples, freeze-drying is preferred over air and oven drying [54]. In some fresh and dried sediments and soil samples, in presence of oxygen and porewater may play a role in methylmercury (MeHg) levels and it can alter even during storage and drying [59]. Furthermore, measures should be taken to avoid the loss of volatile species such as elemental mercury or cause chemical changes for instance precipitation of insoluble species [31].
In the study by Martin et al. [28] 110 soil samples were collected in a regular grid design, with at least 10 soil sub-samples obtained from the top 25 cm of soil at each sampling location. Subsamples were thoroughly mixed in the field. Samples were air-dried and then sieved. In another study, samples for the study come from 22 different locations in Poznan. Using the soil sampler, soil samples were gathered from a 20 cm layer of soil and placed in PE containers. The samples were dried at room temperature before being homogenized and sieved in an agate mortar [60]. In Lake Liinjarv, Estonia, bottom sediment samples were gathered from the lake's lowest depths. A modified Livingstone-Vallentyne piston corer with extension rods was used to retrieve a 62 cm long core of unconsolidated sediments. In the field, the samples were cut into 12 cm thick subsamples, placed into plastic bags, brought to the lab, dried, and crushed to a fine powder [61]. In Koniarz et al. [62] research, an Ekman sampler was used to gather samples from three different places. To average the properties of sediments in samples, 5–6 samples were taken from each zone and mixed. The sediments were collected from the 0–15 cm layer and lastly, all sediment samples were kept in the refrigerator until they were tested. In research was carried out in Wroclaw, from the lawns, soil samples were taken. In ten sites, representative soil samples were taken from the layers 0–5 cm and 5–15 cm inside each of the lawns. Before analysis, the samples were dried and homogenized [63]. Gruba et al. [64], in this study sampling, was done on a regular 4×4 km grid and selected stratified sampling method. Soil samples were air-dried for one week at room temperature before being sieved with a 2 mm sieve.
In Kodamatani et al. [65] research, found that mercury methylation and MeHg demethylation occurred in the soil within days. Also, their results show that freezing soil and sediment samples immediately after collection, followed by freeze-drying, grinding, homogenization, and storing of the dry material in cold, dark conditions until the analysis is the best strategy to preserve MeHg in soil and sediment samples.
Mercury (Hg) is an element that occurs naturally in the earth's crust, and it is the only liquid metal at environmental temperature, therefore also known as quicksilver [41]. The background concentration of Hg in soils is normally between 0.003 and 4.6 mg·kg−1 while contaminated sites have recorded concentrations of up to 11500 and 14000 mg·kg−1 [66].
Mercury species and environmental reactions: There is a wide range of mercury (Hg) species in the world, and bioavailability, transport, persistence, and toxicity can vary in their various chemical types [30]. Mercuric (Hg2+) complexes with different inorganic and organic ligands are the predominant dissolved Hg species in soil systems. Temperature, pH, light, flooding conditions, ionic activity, and redox potential are the main factors influencing the aqueous species of Hg. Besides, Hg can be mobilized in well-oxygenated soil through the presence of high chloride (Cl-) concentrations. Dissolved organic matter (DOM) concentrations, dissolved oxygen, sulfide, and suspended solids may also play a role and Hg strongly interacts with soil organic matter [29,57,68].
Hg can exist in three oxidation states elemental (HgO), monovalent (HgⅠ, Hg22+), divalent (HgⅡ, Hg2+) [29,69,70], and Robles et al. [30] and Saiz-Lopez et al. [71] have defined Hg as elemental (HgO), oxidized inorganic (HgⅠ, HgⅡ, Hg2+-mercuric, Hg22+-mercurous) and oxidized organic (methyl/ethyl mercury) forms. Mercuric ion can be found especially at low pH levels. High solubilities of Hg happen only in very well-oxygenated environments [68].
The type of Hg2+ appears to generate highly stable complexes and organometallic compounds. Between Hg and various ligands, several complexes could be formed. These can be sulfur, namely thiol groups, and sulfides, nitrogen, phosphorous, or carbon. Hg has a low affinity for oxygen ligands [72].
Most of the Hg present in the environment, except in the atmosphere where more than 90 percent is HgO and HgO (56 µg/liter at 25 ℃) is insoluble in water. In the form of inorganic HgⅡ salts or organomercury compounds which can be vary widely in water solubility. Mercuric chloride, mercuric nitrate, mercuric hydroxide and mercuric sulfide, as inorganic compounds, and the organic species monomethyl mercury, monomethyl mercury chloride and monomethyl mercuric hydroxide are the compounds most likely to be detected under environmental conditions. In biogeochemical processes of transformation, any type of Hg in the environment may grow into a more toxic species (Figure 1) [29,30,68].
Atmospheric HgO deposition to soil occurs over broad time and space, while atmospheric Hg2+ is always easily disposed of by wet or dry deposition to the soil [31]. Flooding can contribute to soil erosion and Hg release to the ecosystem, while increased precipitation will result in greater deposition of Hg from the atmosphere [41].
Usually, monomethyl-mercury accounts for less than 10 percent of the THg, microbial methylation or abiotic processes may help to produce it. Under anaerobic conditions and relatively low pH, bacterial methylation rates tend to increase this process. HgO can be produced by reducing HgO in humic acid. For Hg and MeHg binding to DOM, the sulfur-containing functional groups are mainly considered essential [30,69].
The solubility of HgO and cinnabar (ore) (HgS) is incredibly low, which leads to restrictions between phases by dissolution. However, oxidation increases the solubility of Hg, which is oxidized by ozone, halogens and some components of acid rains in soils. The ozone in air if does not consume by the other more reactive air contaminants, such as consumption by sulfur dioxide, and hydrocarbons, can oxidize Hg to HgⅡ. These natural minerals are not considered to be mobile sources of Hg in the environment, but the environmental risk increases if they are converted into more soluble forms through natural processes. Dissolved Hg appears as HgOH+, HgOHCl, Hg(OH)2, and HgCl2 molecules under less acidic conditions, and as complex anions HgCl42- in high chloride concentrations [30]. Additionally, the existence of salt is also critical for the oxidation of HgO to HgⅡ [34].
While not generally soluble if Hg is dissolved it is either leached into water sources or discharged directly into the atmosphere from mercury polluted soils. Dissolved Hg becomes uniformly distributed in rivers and can contribute to further leaching during soil processes [73]. Also, In the event of chlorinated organic solvents, the confined volume is related to non-aqueous phase liquid (NAPL) saturation or residual organic saturation. A residual liquid can cause extensive groundwater contamination by dissolution [74].
Soil and sediments particle characteristics, such as mineral composition, the quality of clay and organic matter affect Hg sorption. Due to their high adsorption affinity for Hg, the sorption of Hg to natural soil and mineral surfaces is influenced by particulate organic carbon (POC) and dissolved organic carbon (DOC) [75]. Organic matter in soil has a strong affinity for Hg2+. As a result, increased THg concentration levels are frequently linked with organically rich soils, such as forest soils, peaty soils, or rice paddy fields. Soil clay may be a sorbent organic matter. It is proposed that increased Hg sorption capacity in clay soil is associated with the binding of organic material. Hg may create clay-structured covalent connections with oxygen atoms in organic matter functional groups, and it can react with sulfides to generate very stable HgS mineral HgS. It has been observed that the ability of clay Hg sorption can reach 1000 mg for each 1 cmolc per kilogram of soil. Furthermore, Hg is immobilized more effectively than most other elements which can be toxic in soil [31].
In the particulate and dissolved stages, the sorption capability of Hg2+ is highest for organic matter. Sorption of Hg to DOM is monitored by a small fraction of DOM molecules containing reactive thiol functional groups under conditions of low Hg contamination. Oxides, hydroxides, Iron/Manganese/Aluminum oxyhydroxides, amorphous Iron sulfide (FeS) under reduction conditions, and clay minerals are other possible sorbents [29]. Various mineral surface characteristics and bindings for HgⅡ may explain the observed variations in HgⅡ sorption affinity and capability on particulates [76].
In the soil profile, Hg sorption to colloidal organic matter, such as fulvic acids and hydrophilic compounds, can increase the mobility of Hg. In porewater, these species can easily be suspended and transported [31]. Sas-Nowosielska et al. [33] study show that Hg binding to humic and fulvic acids fractions, as well as organic/sulfide bound beyond 30 and 100 mg∙kg-1, confirms that Hg binding to humic substances is the major process in Hg sorption.
For immobilizing soil pollutants through root absorption and accumulation, root adsorption, or root zone precipitation, some plant species have been used [77]. After root absorption, through the transpiration stream, Hg can be transported to the aboveground portion of the plant and eventually show a certain concentration. Plants can play a significant role in the transfer and accumulation of Hg. The capacity of some plants for soil phytoremediation of Hg has been documented. Solanum nigrum (BR3) and Cynodon dactylon (BR2), for example, have greater MeHg concentrations in their biomass [78]. Absorption of organic and inorganic Hg is poor by plants and there is a barrier to translocation of Hg from plant roots to aerial part. Furthermore, plants store Hg compounds mostly in their roots. As a result, significant increases in soil Hg only cause minor increases in plant Hg by direct soil intake [33,79].
The adsorption rate depends primarily on the initial concentration of Hg2+, OM content, and ions in the solution [29]. Sulfide (S-) and Cl- ions and dissolved organic carbon (DOC) are three of the most influential species not only because of their abundance but also for their high stability with Hg [70]. The adsorption of Hg2+ to inorganic and organic adsorbents are reduced by a rise in Cl- concentration. A covalent bond with Hydroxide (OH-) is also created by Hg, which also minimizes the bond between the mineral surface (oxide) and OH- [29]. Two dissolved species, Cl- and DOC, are known to readily break the HgS bond. It has been discovered that hydrophobic components of dissolved OM (a combination of humic and fulvic acids) are more efficient than hydrophilic components in releasing mercury from cinnabar, furthermore, it has been demonstrated that the presence of Ca2+ substantially limits mercury release from HgS by OM [34]. Also, DOC complexes affect Hg adsorption [29,70].
Inorganic colloids contribute more to organomercurials adsorption while inorganic Hg compounds are stronger and large amounts of Hg can attach to soil organic matter. In addition, organic components were also more important at higher Hg concentrations in the adsorption of Hg. In contrast with mineral colloids, this is due to a greater adsorption capacity of OM [68].
Adsorption of Hg also is affected by the soil pH and mineral components are more efficient sorbents in neutral to alkaline soils. As with all metals, adsorption typically declines with a lower, more acidic pH. However, Hg adsorption to humic matter is known to increase at lower pH levels [29]. The adsorption of Hg in bottom sediments that do not contain salt is influenced by the pH of the sediments [34]. Seo et al. [80], explored the sorption potential of mercury and six other elements in a wetland soil was investigated. The seven metals were sorted by adsorptive capacity (mg/g) in batch mono-metal studies at pH = 6, Pb (25.4) ≫ Hg (6.4) > Cr (4.9) > Cd (2.9) ⩾ Cu (2.6) ⩾ Zn (2.4) ≫ As (0.8), respectively. As a result, it was clear that Hg's adsorption capacity remained high in the presence of the other metals, but it was drastically less than Pb.
The soil Hg concentrations, atmospheric Hg concentration, meteorological factors (atmospheric pressure, air temperature, wind speed and turbulence, solar radiation, snow cover), soil moisture content, soil temperature, soil surface characteristics and air mixing characteristics are all factors that affect Hg release from soils [31]. The specific depth of the soil layers that contribute to evaporation, depends on soil profile, formation of volatile Hg species, physical migration of Hg species, and physical and chemical sorption of Hg vapor [81]. The most observed Hg reaction in the soil is the reduction of Hg2+ to HgO [70] and this is the first step in the volatilization of Hg from soil to the atmosphere [29].
Sondreal et al. [82] indicated that one of the sources of Hg is coal-burning plants and 45 % of the anthropogenic THg emissions are related to fossil fuel combustion [83]. There is a significant amount of elemental Hg in the high-temperature gases associated with fossil fuel combustion and only a small portion of Hg is in the oxidized form. Because of this, coal-fired energy production facilities, cement kilns, and high-temperature incinerators as well as chlor-alkali industries have trouble with Hg capture, thus contributing to atmospheric Hg [30]. Pogrzeba et al. [84] indicate that soil from Chlor-alkali plants emits large amounts of Hg into the atmosphere, emphasizing the necessity of adding sulphur to the soil as well as establishing a plant cover to reduce Hg flow to roughly 70% to 80% compared to unplanted areas. Forest fires, which are becoming larger and more prevalent due to anthropogenic climate change, are another source of Hg volatilization and contribute to the mobilization of Hg from soils [85].
Hg reduction in the soil can be biotic or abiotic, and it happens more frequently in lower or saturated strata, which are typically reducing conditions. Reducers such as dissolved organic materials (humic and fulvic acids) or Fe2+ can help in the abiotic reduction of Hg. The pH, dissolved oxygen concentration, and chloride levels all affect Hg reduction by humic and fulvic acids. Adsorption influences abiotic reduction because free Hg2+ in solution has a higher reduction potential than adsorbed Hg compounds. Abiotic reduction of Hg can be caused by photochemical reactions in the first few millimeters of soil. Various bacteria can mediate the biotic reduction of Hg2+ to HgO such as Shewanella oneidensis MR-1 and Geobacter spp. Mercury volatilization, for example, decreases with rising DOM/Cl- content and decreasing moisture content [29].
Hg volatilization is more common where mobile Hg forms predominate than where insoluble HgS predominates [84]. Evaporated oxidized sources of Hg are thought to only contaminate the local environment, but since oxidized Hg sources can be converted to HgO they can also become part of the global Hg cycle. Methylated Hg compounds, which are highly volatile, also are easily released into the atmosphere [30].
The net result of MeHg production and demethylation is MeHg in the environment. These processes are mostly microbially related [86] and such as those of flooded soil, sediments, anoxic water columns and suspended particles of aquatic systems mainly happen under oxygen-deficient conditions and boreal wetlands, organic-rich riparian soil, and soil that have recently become waterlogged soil are common forest ecosystems with elevated MeHg formation [87,88].
The presence of nutrients also can play a role in the higher methylation rates found in sediments versus in the water column. This may be related to higher nutrients and carbon content of sediments [68]. It has been proven that OM promotes HgⅡ methylation [1].
MeHg in soil/sediments are difficult to determine since inorganic Hg concentrations are typically 100–1000 times higher than MeHg concentrations, and soil/sediments sample compositions are widely dependent on the site and climate [89].
Soil moisture content has a significant impact on methylation, otherwise, the mechanisms of microbial methylation in soil and sediment are very similar. As moisture increases, water-saturated micropores also increase in the soil. MeHg is produced primarily by sulfate-reducing bacteria (SRB) such as Clostridium butyricum, Desulfobulbus propionicus, Desulfovibrio desulfuricans, Desulfococcus multivorans, Desulfobacter sp., Desulfobacterium sp., iron-reducing bacteria, methanogenic and other microbes as a cometabolic product [34,57,86,90]. In a reduced environment, this allows both sulfate and iron-reducing bacteria that could boost Hg methylation. Soil/sediments temperature also affects the activity of the current microbial community, and the methylation of Hg can be varied seasonally. Yang et al. [91] study show the effects of warming on MeHg production in an Arctic soil during an 8-month anoxic incubation experiment. In that soil, warming from 2 to 8 ℃ resulted in a 10-fold increase in net MeHg production. Study findings suggest that climate change and permafrost thawing could boost MeHg production and lastly, significant correlations have been found between mercury methylation and the formation of methane and ferrous ions. With climate change, Hg methylation can be increased in sediments at warm temperatures, which could result in higher concentrations of bioavailable MeHg [92]. Increased concentrations of MeHg are also associated with increased concentrations of sulfates. Dissolved organic carbon has been demonstrated to increase Hg methylation by inducing microbial activity [72]. Organic Hg and total organic carbon, as well as organic Hg and elemental Hg content, were found to have positive relations. These findings indicate that elemental Hg in the soil can be converted to reactive HgⅡ, primarily by oxidation, and then methylated by microbial activity [73]. However, the formation of MeHg in the ecosystem will be governed by a variety of factors regulating microbial activity and/or the geochemical speciation of inorganic HgⅡ [87]. According to Dang et al. [93], Selenium (Se) has also an impact on MeHg production via HgSe formation in soil and sediments.
Demethylation of MeHg is a reverse method of methylation of Hg. It may also proceed by biotic pathways or abiotic pathways, like methylation of Hg [73,90]. The major pathway of Hg demethylation in sediments and periphyton has been proposed to be the biotic process [94]. In summer, MeHg demethylation in sediments typically increases. Significant parameters that also control the processes of Hg demethylation are sediment's redox potential and often its association with Hg concentration [72]. Additionally, a laboratory incubation analysis of surface lake sediments in China found faster demethylation and slower methylation of Hg at higher concentrations of solid-phase Se and that pH effect was variable due to the availability of Hg and microbial activity [87].
Degradation of MeHg by bacteria primarily requires reduction of Hg2+ to HgO and under aerobic conditions seems to be preferred. Natural demethylation is normally activated by either microbial activity or photoreduction of light. Two forms of microbial demethylation reactions typically exist: reductive and oxidative. Reductive demethylation degrades MeHg into HgO and methane (CH4), and HgO and carbon dioxide (CO2) are formed by oxidative demethylation [29].
The deposition of Hg in surface soil under anaerobic and low pH conditions can be affected the formation of net MeHg and Hg methylation rate in soil may therefore also be a result of the concentration and deposition processes of atmospheric Hg [31]. Boszke et al. [34] indicate that low pH promotes the release of mercury from bottom sediments, whereas others argue that low pH enhances mercury sorption on the sediments.
The analysis is a sequential process involving extraction/pre-concentration, separation, and detection. Extraction/pre-concentration methods are liquid phase microextraction, purge and trap, solid-phase extraction, and solid-phase microextraction. Separation techniques are Chromatographic techniques and non-chromatographic techniques [54].
Most of the methods for the measurement of THg in solid samples require preliminary digestion. In an acidic medium to release Hg from the sample, nitric acid (HNO3), hydrochloric acid (HCl), bromine monochloride (BrCl), sulfuric acid (H2SO4), perchloric acid (HClO4), hydrogen peroxide (H2O2), vanadium pentoxide (V2O5), potassium permanganate (KMnO4), potassium dichromate (K2Cr2O7) are the most common reagents which have been used. Samples are normally digested at high temperatures, such as max. 90–100℃, in closed, semi-closed, or sealed containers. At high digestion temperatures, careful attention should be paid to preventing Hg loss. Thus, closed, or sealed digestion containers should be applied [29]. Soil samples are digested with aqua regia (HCl/HNO3) using microwave-assisted digestion for the extraction of pseudo-THg, due to its capacity to dissolve HgS. Soil samples can be digested in HNO3/H2SO4 containing large quantities of organic material and then diluted with BrCl solution to eliminate any remaining organic material [31]. For solid samples such as soil and sediments, lower and inconsistent results are provided by acid digestion using aqua regia. The use of acid digestion, including hydrofluoric acid (HF), is highly recommended to fully extract Hg from the inorganic matrix [29].
It is important to isolate MeHg from the sample before analysis to prevent matrix interference during sample processing [95]. Acid extraction (mostly combined with solvent extraction), distillation, and alkaline extraction are the most widely used methods for removing organomercury species from environmental samples [59]. The derivatization (chemical changes) steps needed for gas chromatography (GC) analysis are especially sensitive to matrix suppression. To remove MeHg from complex matrices, distillation methods are commonly used. This technique is based on a simple distillation of atmospheric pressure and does not require complex reagents for accurate results to be obtained. However, the use of distillation may lead to the formation of an artifact of MeHg [95].
Extraction of soil samples for MeHg analysis is more difficult. Extraction can include digestion to extract organic Hg from inorganic complexes with acidic potassium bromide (KBr) and copper sulfate (CuSO4) solution, followed by dichloromethane (DCM) to extract MeHg, and then back-extraction by argon (Ar) purging into an aqueous solution. In a purge vessel using sodium tetraethyl borate, aqueous solutions are ethylated to convert MeHg to volatile methylethyl-Hg species [31].
Usually, extremely sensitive atomic absorption and atomic emission techniques assess only the total quantity of metal in the sample and must therefore be combined with a chromatographic or other separation technique until it is possible to classify individual species [96]. By using different methods, the organo-Hg material can then be detected [31]. High-performance liquid chromatography-chemiluminescence (HPLC-CL), electron capture gas chromatography (GC-ECD), and gas chromatography-atomic fluorescence spectrometry (GC-AFS), for these methods, MeHg results for all soil and sediments samples returned comparable. Although, it was reported that the HPLC-CL method performed poorly in analyzing high sulfur content samples [65]. Also, Due to its speed, simplicity, relative freedom from interference, low operating costs, and high sensitivity, especially when Hg vapor is pre-concentrated on gold by amalgamation, cold vapor atomic absorption spectrometry (CV-AAS) has been used. Many published methods have relied on plasma mass spectrometry that is inductively coupled (ICP-MS) [97].
Denmark et al. [98] developed a new method for extracting MeHg selectively from severely contaminated soil and sediment samples contaminated with Hg for analysis using chemical vapor generation inductively coupled plasma mass spectrometry (CVG-ICP-MS). HNO3 was shown to be the most effective for selective extraction of MeHg from soils when compared to HCl. Fast extraction of MeHg was achieved using ultrasonic agitation in HNO3 at room temperature. Using a dilute ammonium sulfide solution, soil extracts in HNO3 were used to precipitate HgS, which resulted in the elimination of all residual Hg2+ without affecting MeHg levels. Compared to chromatographic separation and speciation methods, the procedure is simple and quick, and it also has distinct advantages for determining trace levels of very dangerous MeHg from severely Hg-contaminated sediments and soil. In another recent study, Saniewska and Beldowska [99], tried to develop a simple thermo-desorption method for mercury fractionation in soil and sediment samples using a direct mercury analyzer and they used soil, beach sand and marine sediment in this study. Then, the temperature range in which mercury species were released was used to identify them. Despite some limitations, the results suggest that temperature fractionation can be used as a screening approach for determining the percentage contribution groups of Hg compounds with similar properties in solid materials. This approach could be used on solid samples with low levels of Hg in the environment. Lastly, in 2013, Kodamatani and Tomiyasu [89], developed simultaneous determination of MeHg and ethylmercury (EtHg). The approach involves extracting MeHg and EtHg into toluene as chlorides after eluting mercury species from soil/sediment samples with HCl containing Cu2+ and Pd2+. These alkylmercury chlorides were then back-extracted into an aqueous EDTA solution, forming EDTA complexes, which were subsequently separated using reverse-phase HPLC and identified using a tris ruthenium chemiluminescence process.
Anthropogenic emissions of Hg have a vast effect on the environment and therefore, pose an elevated risk to human beings. This overview aims to disclose sampling methods, use and storage; it also focuses on Hg forms, mobilization and analysis in soil and sediment.
The findings of this study are listed below:
• In the sampling method, different approaches depend on the chosen area, such as two-stage, cluster, judgmental, random, stratified random, systematic grid, search and transect. Tools made of polymer, glass, stainless steel, or aluminum are required during the collecting, pre-treatment, and storage phases of environmental samples. The best technique for preserving MeHg is to freeze soil and sediment samples immediately after collection, followed by freeze-drying, grinding, homogenization, and storing the dry material in cold, dark conditions until analysis. In most of the papers, it is not specified what type of sampling or drying methods were applied.
• Clay soils can absorb Hg and lead the creation of HgS.
• Organically rich soils, such as forest soils, peaty soils, or rice paddy fields are typically connected to higher THg concentrations.
• Hg binding to humic substrates is the major process in Hg sorption.
• Plants can play an important role in Hg transport and accumulation. MeHg concentrations in biomass were found to be higher in Solanum nigrum (BR3) and Cynodon dactylon (BR2).
• It appeared that the adsorptive capacity of Hg is higher than Cr, Cd, Cu, Zn and As but lower than Pb.
• Hg can be affected by most of the conditions in the environment, which can determine the final form in soil or sediment, such as pH, redox potential, and light.
• The soil profile, production of volatile Hg species, physical movement of Hg species, and physical and chemical sorption of Hg vapor all influence the depth of the soil layers that contribute to evaporation. When compared to unplanted regions, applying sulfur to the soil and growing a plant cover reduces Hg flow by around 70% to 80%.
• Shewanella oneidensis MR-1 and Geobacter spp are two bacteria that can mediate the biotic reduction of Hg2+ to HgO.
• The most common organic and toxic form of Hg in the environment is accepted as MeHg. Soil moisture highly impacts MeHg, through sulfate and iron-reducing bacteria.
• A laboratory incubation analysis of surface lake sediments revealed that higher levels of solid-phase Se resulted in rapid demethylation and slower methylation of Hg and that the pH impact was varied owing to Hg availability and microbial activity.
• Climate change and permafrost thawing have the potential to increase MeHg production. Arctic permafrost represents an important source of Hg in case warming will not decrease in the future.
• In 2018, a simple and quick approach for analyzing MeHg utilizing chemical vapor generation inductively coupled plasma mass spectrometry was introduced. When compared to HCl, HNO3 has proven to be the most effective for selective extraction of MeHg from soils. Ultrasonic agitation helped to produce rapid MeHg extraction.
All authors declare no conflicts of interest in this paper.
[1] | Skrobonja A (2019) Formation, uptake, and bioaccumulation of methylmercury in coastal seas - a Baltic Sea case study. Doctoral Dissertation, Umea University. http://umu.diva-portal.org/ |
[2] |
Kwasigroch U, Bełdowska M, Jędruch A, et al. (2021) Distribution and bioavailability of mercury in the surface sediments of the Baltic Sea. Environ Sci Pollut Res 28: 35690-35708. https://doi.org/10.1007/s11356-021-13023-4 doi: 10.1007/s11356-021-13023-4
![]() |
[3] |
Siedlewicz G, Korejwo E, Szubska M, et al. (2020) Presence of mercury and methylmercury in Baltic Sea sediments, collected in ammunition dumpsites, Marine Environmental Research 162: 105158. https://doi.org/10.1016/j.marenvres.2020.105158 doi: 10.1016/j.marenvres.2020.105158
![]() |
[4] | Manzetti S (2020) Heavy metal pollution in the Baltic Sea, from the North European coast to the Baltic states, Finland and the Swedish coastline to Norway, Doctoral Dissertation. https://doi.org/10.13140/RG.2.2.11144.85769/1 |
[5] |
Bełdowska M, Saniewska D, Falkowska, L (2014) Factors influencing variability of mercury input to the Southern Baltic Sea. Mar Pollut Bull 86: 283-290. http://doi:10.1016/j.marpolbul.2014.07.004 doi: 10.1016/j.marpolbul.2014.07.004
![]() |
[6] | Kocman D, Horvat M, Pirrone N, et al. (2013) Contribution of contaminated sites to the global mercury budget. Environ. Res. 125: 160-170. https://doi.org/10.1016/j.envres.2012.12.011 |
[7] |
Panagos P, Jiskra M, Borrelli P, et al. (2021) Mercury in European topsoils: Anthropogenic sources, stocks and fluxes. Environmental Research 201: 111556. https://doi.org/10.1016/j.envres.2021.111556 doi: 10.1016/j.envres.2021.111556
![]() |
[8] | SedNet, Objectives of Sediment and its management, 2021. Available From: https://sednet.org/about/objectives/. |
[9] |
Li C, Quan Q, Gan Y, et al. (2020) Effects of heavy metals on microbial communities in sediments and establishment of bioindicators based on microbial taxa and function for environmental monitoring and management. Science of The Total Environment 749: 141555. https://doi.org/10.1016/j.scitotenv.2020.141555 doi: 10.1016/j.scitotenv.2020.141555
![]() |
[10] |
Szymczycha B, Miotk M, Pempkowiak J (2013) Submarine Groundwater Discharge as a Source of Mercury in the Bay of Puck, the Southern Baltic Sea. Water Air Soils Pollut 224: 1542. https://doi.org/10.1007/s11270-013-1542-0 doi: 10.1007/s11270-013-1542-0
![]() |
[11] |
Leipe T, Moros M, Kotilainen A, et al. (2013) Mercury in Baltic Sea sediments-Natural background and anthropogenic impact, Geochemistry 73: 249-259. https://doi.org/10.1016/j.chemer.2013.06.005 doi: 10.1016/j.chemer.2013.06.005
![]() |
[12] | Helcom (2018) State of the Baltic Sea - Second HELCOM holistic assessment 2011-2016. Baltic Sea Environment Proceedings 155: 1-155. |
[13] |
Bełdowska M, Jędruch A, Łęczyński L, et al. (2016) Coastal erosion as a source of mercury into the marine environment along the Polish Baltic shore. Environ Sci Pollut Res 23: 16372-16382. https://doi.org/10.1007/s11356-016-6753-7 doi: 10.1007/s11356-016-6753-7
![]() |
[14] |
Careghini A, Mastorgio A, Saponaro S, et al. (2015) Bisphenol A, nonylphenols, benzophenones, and benzotriazoles in soils, groundwater, surface water, sediments, and food: a review. Environ Sci Pollut Res 22: 5711-5741. https://doi.org/10.1007/s11356-014-3974-5 doi: 10.1007/s11356-014-3974-5
![]() |
[15] |
Peng W, Li X, Xiao S, et al. (2018) Review of remediation technologies for sediments contaminated by heavy metals. J Soils Sediments 18: 1701-1719. https://doi.org/10.1007/s11368-018-1921-7 doi: 10.1007/s11368-018-1921-7
![]() |
[16] | Boszke L, Kowalski A (2006) Spatial Distribution of Mercury in Bottom Sediments and Soils from Poznan, Poland. Pol J Environ Stud 15: 211-218. |
[17] |
Wada SI (2002) Effect of clay mineralogy on the feasibility of electrokinetic soil decontamination technology. Applied Clay Science 20: 283-293. https://doi.org/10.1016/S0169-1317(01)00080-1 doi: 10.1016/S0169-1317(01)00080-1
![]() |
[18] |
Shahidi D, Roy R, Azzouz A (2015) Advances in catalytic oxidation of organic pollutants - Prospects for thorough mineralization by natural clay catalysts. Applied Catalysis B: Environmental 174: 277-292. https://doi.org/10.1016/j.apcatb.2015.02.042 doi: 10.1016/j.apcatb.2015.02.042
![]() |
[19] |
Kern K, Loffelsend T (2004) Sustainable development in the Baltic Sea region. Governance beyond the nation state. Local Environment 9: 451-467. https://doi.org/10.1080/1354983042000255351 doi: 10.1080/1354983042000255351
![]() |
[20] |
Ullrich S, Tanton T, Abdrashitova S (2001) Mercury in the Aquatic Environment: A Review of Factors Affecting Methylation. Critical Reviews in Environmental Science and Technology 31: 241-293. https://doi.org/10.1080/20016491089226 doi: 10.1080/20016491089226
![]() |
[21] | EEA (European Environment Agency) (2000) Down to Earth: soils degradation and sustainable development in Europe. Environmental issues series 16: 32. |
[22] | EEA (European Environment Agency) (2001) Proposal for a European soils monitoring and assessment framework. Environmental issues series 61: 58. |
[23] |
Ramos-Miras JJ, Gil C, Rodriguez Martin JA, et al. (2020) Ecological risk assessment of mercury and chromium in greenhouse soils. Environ Geochem Health 42: 313-324. https://doi.org/10.1007/s10653-019-00354-y doi: 10.1007/s10653-019-00354-y
![]() |
[24] |
Lado L, Hengl Y, Reuter H (2008) Heavy metals in European soils: A geostatistical analysis of the FOREGS Geochemical database. Geoderma 148: 189-199. https://doi.org/10.1016/j.geoderma.2008.09.020 doi: 10.1016/j.geoderma.2008.09.020
![]() |
[25] | Long Q, Wang JY, Da LJ (2013) Assessing the spatial‐temporal variations of heavy metals in farmland soil of Shanghai, China. Fresenius Environmental Bulletin 22: 928-938. |
[26] |
Ballabio C, Jiskra M, Osterwalder S, et al. (2021) A spatial assessment of mercury content in the European Union topsoil. Science of The Total Environment 769: 144755. https://doi.org/10.1016/j.scitotenv.2020.144755. doi: 10.1016/j.scitotenv.2020.144755
![]() |
[27] | Medyńska-Juraszek A, Kabala C (2010) Lead, mercury, and cadmium in forest soils impacted by copper smelting in south-west Poland. 15th International Conference on Heavy Metals in the Environment 2010: 19-23. |
[28] |
Martin JAR, Gutierrez C, Escuer M, et al. (2021) Trends in soil mercury stock associated with pollution sources on a Mediterranean island (Majorca, Spain). Environmental Pollution 283: 117397. https://doi.org/10.1016/j.envpol.2021.117397. doi: 10.1016/j.envpol.2021.117397
![]() |
[29] | Horvart M, Kotnik J (2019) Technical information report on mercury monitoring in soils. UN Environment. Chemicals and Health Branch Switzerland 2019: 54. |
[30] | Robles, I, Lakatos, J, Scharek, P (2014) Remediation of Soils and Sediments Polluted with Mercury: Occurence, Transformations, Environmental Consideration and San Joaquin's Sierra Gorda Case, InTech, 35-75. |
[31] |
O'Connor D, Hou D, Ok Y, et al. (2019) Mercury speciation, transformation, and transportation in soils, atmospheric flux, and implications for risk management: A critical review. Environment International 126: 747-761. https://doi.org/10.1016/j.envint.2019.03.019 doi: 10.1016/j.envint.2019.03.019
![]() |
[32] |
Jędruch A, Falkowska L, Saniewska D, et al. (2021) Status and trends of mercury pollution of the atmosphere and terrestrial ecosystems in Poland. Ambio 50: 1698-1717. https://doi.org/10.1007/s13280-021-01505-1 doi: 10.1007/s13280-021-01505-1
![]() |
[33] |
Sas-Nowosielska A, Galimska-Stypa R, Kucharski R, et al. (2008) Remediation aspect of microbial changes of plant rhizosphere in mercury contaminated soils. Environ Monit Assess 137: 101-109. https://doi.org/10.1007/s10661-007-9732-0 doi: 10.1007/s10661-007-9732-0
![]() |
[34] | Boszke L, Kowalski A, Glosinska G. et al. (2003) Environmental factors affecting speciation of mercury in the bottom sediments; an overview. Polish Journal of Environmental Studies 12: 5-13. |
[35] |
Pacyna E, Pacyna J, Pirrone N (2001) European emissions of atmospheric mercury from anthropogenic sources in 1995. Atmospheric Environment 35: 2987-2996. https://doi.org/10.1016/S1352-2310(01)00102-9 doi: 10.1016/S1352-2310(01)00102-9
![]() |
[36] |
Glodek A, Panasiuk D, Pacyna J (2010) Mercury Emission from Anthropogenic Sources in Poland and Their Scenarios to the Year 2020. Water Air Soil Pollut 213: 227-236. https://doi.org/10.1007/s11270-010-0380-6 doi: 10.1007/s11270-010-0380-6
![]() |
[37] | Bartnicki J, Gusev A, Aas W, et al. Atmospheric Supply of Nitrogen, Lead, Cadmium, Mercury and Dioxins/Furans to the Baltic Sea in 2005. EMEP, 2007. Available from: https://emep.int/publ/helcom/2007. |
[38] | Ministry of Environment of the Republic of Lithuania National environmental protection strategy, 2016. Available from: https://am.lrv.lt/uploads/am/documents/files/National%20Environmental%20Protection%20Strategy.pdf. |
[39] |
Šakalys J, Kvietkus K, Garbarienė I, et al. (2019) Long-term study of atmospheric mercury deposition at monitoring stations in Lithuania. Lithuanian Journal of Physics 59: 1. https://doi/10.3952/physics.v59i1.3940 doi: 10.3952/physics.v59i1.3940
![]() |
[40] | Science for Environment Policy (2017) Tackling mercury pollution in the EU and worldwide. In-depth Report 15 produced for the European Commission. DG Environment by the Science Communication Unit. |
[41] |
EEA (European Environment Agency) (2018) Mercury in Europe's environment A priority for European and global action. Environmental issues series 11: 72. https://doi/10.2800/558803 doi: 10.2800/558803
![]() |
[42] |
Schuster P, Schaefer K, Aiken G, et al. (2018) Permafrost stores a globally significant amount of mercury. Geophysical Research Letters 45: 1463-1471. https://doi.org/10.1002/2017GL075571 doi: 10.1002/2017GL075571
![]() |
[43] |
Artiola J, Brusseau M. (2019) 10 - The Role of Environmental Monitoring in Pollution Science, Environmental and Pollution Science (Third Edition), Academic Press 2019: 149-162. https://doi.org/10.1016/C2017-0-00480-9 doi: 10.1016/C2017-0-00480-9
![]() |
[44] |
Artiola J, Pepper I, Brusseau M (2004) 1 - Environmental Monitoring and Characterization. Environmental Monitoring and Characterization. Elsevier Academic Press 2004: 1-9. https://doi.org/10.1016/B978-0-12-064477-3.X5000-0 doi: 10.1016/B978-0-12-064477-3.X5000-0
![]() |
[45] |
Loveland P, Bellamy P (2005) Environmental Monitoring. Encyclopedia of Soils in the Environment. Elsevier 2005: 441-448. https://doi.org/10.1016/B0-12-348530-4/00092-8 doi: 10.1016/B0-12-348530-4/00092-8
![]() |
[46] |
Morvan X, Saby NPA, Arrouays D, et al. (2008) Soil monitoring in Europe: A review of existing systems and requirements for harmonisation. Science of The Total Environment 391: 1-12. https://doi.org/10.1016/j.scitotenv.2007.10.046 doi: 10.1016/j.scitotenv.2007.10.046
![]() |
[47] |
Van Leeuwen E, Saby N, Jones A, et al. (2017) Gap assessment in current soils monitoring networks across Europe for measuring soils functions. Environ Res Lett 12: 124007. https://10.1088/1748-9326/aa9c5c doi: 10.1088/1748-9326/aa9c5c
![]() |
[48] | Brils J (2008) Sediments monitoring and the European Water Framework Directive. Annali dell'Istituto superiore di sanita 44: 218-223. |
[49] | Reuther R (2009) Lake and river sediments monitoring. Environmental Monitoring. Encyclopedia of Life Support Systems Ⅱ 2009: 9. |
[50] | SedNet Sediments Management-an essential element of River Basin Management Plans 28 pages, 2006. Available From: https://sednet.org/download/061122_Report_SedNet_Round_Table_Discussion.pdf. |
[51] |
Ramsey C (2015) Considerations for Sampling Contaminants in Agricultural Soils. Journal of AOAC International 98: 309-315. https://doi.org/10.5740/jaoacint.14-268 doi: 10.5740/jaoacint.14-268
![]() |
[52] | International atomic energy agency (IAEA) (2004) Soils Sampling for Environmental Contaminants, IAEA-TECDOC-1415. Technical Reports Series, Vienna. |
[53] | International atomic energy agency (IAEA) (2019) Guidelines on Soils and Vegetation Sampling for Radiological Monitoring. Technical Reports Series 486, IAEA, Vienna. |
[54] |
Amde M, Yin Y, Zhang D. et al. (2016) Methods and recent advances in speciation analysis of mercury chemical species in environmental samples: a review. Chemical Speciation & Bioavailability 28: 51-65. https://doi.org/10.1080/09542299.2016.1164019 doi: 10.1080/09542299.2016.1164019
![]() |
[55] |
Yu L, Yan X (2003) Factors affecting the stability of inorganic and methylmercury during sample storage. TrAC Trends in Analytical Chemistry 22: 245-253. https://doi.org/10.1016/S0165-9936(03)00407-2 doi: 10.1016/S0165-9936(03)00407-2
![]() |
[56] | Diederick J (2013) Literature review on mercury speciation soils systems under oxidizing conditions. Snowman Network, Project No. SN-03/08. |
[57] |
Gilli R, Claudine K, Mischa W, et al. (2018) Speciation and Mobility of Mercury in Soils Contaminated by Legacy Emissions from a Chemical Factory in the Rhô ne Valley in Canton of Valais. Switzerland Soils Syst 2: 44. https://doi.org/10.3390/soilsystems2030044 doi: 10.3390/soilsystems2030044
![]() |
[58] |
Kodamatani H, Balogh S, Nollet Y, et al. (2016) An inter-laboratory comparison of different analytical methods for the determination of monomethylmercury in various soils and sediments samples: A platform for method improvement. Chemosphere 169: 32-39. https://doi.org/10.1016/j.chemosphere.2016.10.129 doi: 10.1016/j.chemosphere.2016.10.129
![]() |
[59] |
Leermakers M, Baeyens W, Quevauviller P, et al. (2005) Mercury in environmental samples: Speciation, artifacts and validation. TrAC Trends in Analytical Chemistry 24: 383-393. https://doi.org/10.1016/j.trac.2004.01.001 doi: 10.1016/j.trac.2004.01.001
![]() |
[60] |
Kowalski A, Frankowski M (2016) Seasonal variability of mercury concentration in soils, buds and leaves of Acer platanoides and Tilia platyphyllos in central Poland. Environ Sci Pollut Res 23: 9614-9624. https://doi.org/10.1007/s11356-016-6179-2 doi: 10.1007/s11356-016-6179-2
![]() |
[61] |
Lepane V, Varvas M, Viitak A, et al. (2007) Sedimentary record of heavy metals in Lake Rõ uge Liinjärv, southern Estonia. Estonian Journal of Earth Sciences 56: 221-232. https://doi.org/10.3176/earth.2007.03 doi: 10.3176/earth.2007.03
![]() |
[62] |
Koniarz T, Tarnawski M, Baran A, et al. (2015) Mercury contamination of bottom sediments in water reservoirs of southern Poland. Biblioteka Glowna 41: 169-175. https://doi.org/10.7494/geol.2015.41.2.169 doi: 10.7494/geol.2015.41.2.169
![]() |
[63] | Dradrach A, Karczewska A (2013) Mercury in soils of municipal lawns in Wroclaw, Połand. Fresenius Environmental Bulletin 22: 968-972. |
[64] |
Gruba P, Socha J, Pietrzykowski M, et al. (2019) Tree species affects the concentration of total mercury (Hg) in forest soils: Evidence from a forest soil inventory in Poland. Science of The Total Environment 647: 141-148. https://doi.org/10.1016/j.scitotenv.2018.07.452 doi: 10.1016/j.scitotenv.2018.07.452
![]() |
[65] |
Kodamatani H, Maeda C, Balogh S, et al. (2017) The influence of sample drying and storage conditions on methylmercury determination in soils and sediments. Chemosphere 173: 380-386 https://doi.org/10.1016/j.chemosphere.2017.01.053 doi: 10.1016/j.chemosphere.2017.01.053
![]() |
[66] |
Arbestain M, Lado L, Bao M, et al. (2009) Assessment of Mercury-Polluted Soils Adjacent to an Old Mercury-Fulminate Production Plant. Applied and Environmental Soils Science 2009: 8. https://doi.org/10.1155/2009/387419 doi: 10.1155/2009/387419
![]() |
[67] |
Leopold K, Foulkes M, Worsfold P (2010) Methods for the determination and speciation of mercury in natural waters- a review. Analytica Chimica Acta 663: 127-138. https://doi.org/10.1016/j.aca.2010.01.048 doi: 10.1016/j.aca.2010.01.048
![]() |
[68] |
Schuster E (1991) The behavior of mercury in the soils with special emphasis on complexation and adsorption processes - A review of the literature. Water, Air, and Soils Pollution 56: 667-680. https://doi.org/10.1007/BF00342308 doi: 10.1007/BF00342308
![]() |
[69] |
Saponaro S, Sezenna E, Bonomo L (2005) Remediation Actions by a Risk Assessment Approach: A Case Study of Mercury Contamination. Water Air Soils Pollut 168: 187-212. https://doi.org/10.1007/s11270-005-1248-z doi: 10.1007/s11270-005-1248-z
![]() |
[70] |
Gabriel M, Williamson D (2004) Principal Biogeochemical Factors Affecting the Speciation and Transport of Mercury through the terrestrial environment. Environmental Geochemistry and Health 26: 421-434. https://doi.org/10.1007/s10653-004-1308-0 doi: 10.1007/s10653-004-1308-0
![]() |
[71] |
Saiz-Lopez A, Travnikov O, Sonke J, et al. (2020) Photochemistry of oxidized Hg(I) and Hg(Ⅱ) species suggests missing mercury oxidation in the troposphere. Proc Natl Acad Sci USA 117: 30949-30956. http://10.1073/pnas.1922486117 doi: 10.1073/pnas.1922486117
![]() |
[72] | Segade S, Teresa D, Elsa R (2011) Mercury methylation versus demethylation: Main processes involved. Methylmercury: Formation, Sources and Health Effects. Nova Science Publishers 7: 123-166. http://hdl.handle.net/10198/6750 |
[73] |
Higueras P, Fernández-Martínez R, Esbrí J, et al. (2015) Mercury Soil Pollution in Spain: A Review. Environment, Energy and Climate Change I 32: 135-158. https://doi.org/10.1007/698_2014_280 doi: 10.1007/698_2014_280
![]() |
[74] |
Devasena M, Nambi I (2010) Migration and entrapment of mercury in porous media. Journal of Contaminant Hydrology 117: 60-70. https://doi.org/10.1016/j.jconhyd.2010.06.005 doi: 10.1016/j.jconhyd.2010.06.005
![]() |
[75] |
Bengtsson G, Picado F (2008) Mercury sorption to sediments: Dependence on grain size, dissolved organic carbon, and suspended bacteria. Chemosphere 73: 526-531. https://doi.org/10.1016/j.chemosphere.2008.06.017 doi: 10.1016/j.chemosphere.2008.06.017
![]() |
[76] |
Zhang L, Wu S, Zhao L, et al. (2019) Mercury Sorption and Desorption on Organo-Mineral Particulates as a Source for Microbial Methylation. Environmental Science & Technology 53: 2426-2433. https://doi.org/10.1021/acs.est.8b06020 doi: 10.1021/acs.est.8b06020
![]() |
[77] |
Tangahu B, Abdullah S, Basri H, et al. (2011) A Review on Heavy Metals (As, Pb, and Hg) Uptake by Plants through Phytoremediation. International Journal of Chemical Engineering 2011. https://doi.org/10.1155/2011/939161 doi: 10.1155/2011/939161
![]() |
[78] |
Li Q, Tang L, Qiu Q, et al. (2020) Total mercury and methylmercury in the soil and vegetation of a riparian zone along a mercury-impacted reservoir. Science of The Total Environment 738: 139794. https://doi.org/10.1016/j.scitotenv.2020.139794 doi: 10.1016/j.scitotenv.2020.139794
![]() |
[79] |
Gworek B, Dmuchowski W, Baczewska-Dąbrowska H (2020) Mercury in the terrestrial environment: a review. Environmental Sciences Europe 32: 128. https://doi.org/10.1186/s12302-020-00401-x doi: 10.1186/s12302-020-00401-x
![]() |
[80] |
Seo DC, Yu K, DeLaune RD (2008) Comparison of monometal and multimetal adsorption in Mississippi River alluvial wetland sediment: batch and column experiments. Chemosphere 73: 1757-1764. https://doi.org/10.1016/j.chemosphere.2008.09.003 doi: 10.1016/j.chemosphere.2008.09.003
![]() |
[81] |
Schluter K (2000) Review: Evaporation of mercury from soils. An integration and synthesis of current knowledge. Environmental Geology 39: 249-271. https://doi.org/10.1007/s002540050005 doi: 10.1007/s002540050005
![]() |
[82] |
Sondreal E.A, Benson S.A, Pavlish J.H, et al. (2004) An overview of air quality Ⅲ. Mercury, trace element and particulate matter. Fuel Processing Technology 85: 425-440. https://doi.org/10.1016/j.fuproc.2004.02.002 doi: 10.1016/j.fuproc.2004.02.002
![]() |
[83] |
Zhao S, Pudasainee D, Duan Y, et al. (2019) A review on mercury in coal combustion process: Content and occurrence forms in coal, transformation, sampling methods, emission and control technologies. Progress in Energy and Combustion Science 73: 26-64. https://doi.org/10.1016/j.pecs.2019.02.001 doi: 10.1016/j.pecs.2019.02.001
![]() |
[84] |
Pogrzeba M, Ciszek D, Galimska-Stypa R, et al. (2016) Ecological strategy for soil contaminated with mercury. Plant and Soil 409: 371-387. https://doi.org/10.1007/s11104-016-2936-8 doi: 10.1007/s11104-016-2936-8
![]() |
[85] |
Caldwell C, Canavan C, Bloom N (2000) Potential effects of forest fire and storm flow on total mercury and methylmercury in sediments of an arid-lands reservoir. Science of The Total Environment 260: 125-133. https://doi.org/10.1016/S0048-9697(00)00554-4 doi: 10.1016/S0048-9697(00)00554-4
![]() |
[86] |
Bigham G, Murray K, Masue-Slowey, et al. (2016) Biogeochemical controls on methylmercury in soils and sediments: Implications for site management: Geochemical Controls on Mercury Methylation. Integrated Environ. Assessment and Management 13: 249-263. https://doi.org/10.1002/ieam.1822 doi: 10.1002/ieam.1822
![]() |
[87] |
Xu J, Buck M, Eklöf K (2019) Mercury methylating microbial communities of boreal forest soils. Sci Rep 9: 518. https://doi.org/10.1038/s41598-018-37383-z doi: 10.1038/s41598-018-37383-z
![]() |
[88] | Tjerngren I, Karlsson T, Björn E, et al. (2012) Potential Hg methylation and MeHg demethylation rates related to the nutrient status of different boreal wetlands. Biogeochemistry 108: 335-350. http://www.jstor.org/stable/41410599 |
[89] |
Kodamatani H, Tomiyasu T (2013) Selective determination method for measurement of methylmercury and ethylmercury in soils/sediments samples using high-performance liquid chromatography-chemiluminescence detection coupled with simple extraction technique. Journal of Chromatography A 1288: 155-159. https://doi.org/10.1016/j.chroma.2013.02.004 doi: 10.1016/j.chroma.2013.02.004
![]() |
[90] |
Strickman R, Mitchell C (2017) Methylmercury production and accumulation in urban stormwater ponds and habitat wetlands. Environmental Pollution 221: 326-334. https://doi.org/10.1016/j.envpol.2016.11.082 doi: 10.1016/j.envpol.2016.11.082
![]() |
[91] |
Yang Z, W Fang, X Lu, et al. (2016) Warming increases methylmercury production in an Arctic soil. Environmental Pollution 214: 504-509. https://doi.org/10.1016/j.envpol.2016.04.069 doi: 10.1016/j.envpol.2016.04.069
![]() |
[92] | Dijkstra J, Buckman K, Ward D, et al. (2013) Experimental and Natural Warming Elevates Mercury Concentrations in Estuarine Fish. PLoS ONE 8: e58401. https://doi.org/10.1371/journal.pone.0058401 |
[93] |
Dang F, Li Z, Zhong H (2019) Methylmercury and selenium interactions: Mechanisms and implications for soil remediation. Critical Reviews in Environmental Science and Technology 49: 1737-1768. https://doi.org/10.1080/10643389.2019.1583051 doi: 10.1080/10643389.2019.1583051
![]() |
[94] |
Li Y, Cai Y (2013) Progress in the study of mercury methylation and demethylation in aquatic environments. Chin Sci Bull 58: 177-185. https://doi.org/10.1007/s11434-012-5416-4 doi: 10.1007/s11434-012-5416-4
![]() |
[95] |
Perez P, Hintelman H, Quiroz W, et al. (2017) Critical evaluation of distillation procedure for the determination of methylmercury in soils samples. Chemosphere 186: 570-575. https://doi.org/10.1016/j.chemosphere.2017.08.034 doi: 10.1016/j.chemosphere.2017.08.034
![]() |
[96] |
Lund W (1990) Speciation analysis—why and how? Fresenius' Journal of Analytical Chemistry 337: 557-564. https://doi.org/10.1007/BF00322862 doi: 10.1007/BF00322862
![]() |
[97] |
Bernalte E, Salmanighabeshi S, Rueda-Holgado F (2015) Mercury pollution assessment in soil affected by industrial emissions using miniaturized ultrasonic probe extraction and ICP-MS. Int J Environ Sci Technol 12: 817-826. https://doi.org/10.1007/s13762-013-0461-3 doi: 10.1007/s13762-013-0461-3
![]() |
[98] |
Denmark I. S, Begu E, Arslan Z, et al. (2018) Removal of inorganic mercury by selective extraction and coprecipitation for determination of methylmercury in mercury-contaminated soils by chemical vapor generation inductively coupled plasma mass spectrometry (CVG-ICP-MS). Analytica chimica acta 1041: 68-77. https://doi.org/10.1016/j.aca.2018.08.049 doi: 10.1016/j.aca.2018.08.049
![]() |
[99] |
Saniewska D, Bełdowska M (2017) Mercury fractionation in soil and sediment samples using thermo-desorption method. Talanta 168: 152-161. https://doi.org/10.1016/j.talanta.2017.03.026 doi: 10.1016/j.talanta.2017.03.026
![]() |
1. | Theophilus C. Davies, An updated review of the salient geomedical aspects of mercury for enhancement of data quality in simulation modelling and other prognostic applications: Africa case descriptions, 2023, 3, 2673-9283, 10.3389/frans.2023.1069678 | |
2. | Elena Lyapina, Ecological and geochemical features of mercury accumulation in coniferous wood in the Altai Region, 2024, 13, 2222-7962, 95, 10.34220/issn.2222-7962/2023.4/19 | |
3. | Guo Yu, Habib Ullah, Balal Yousaf, Krzysztof Pikoń, Vasileios Antoniadis, Majeti Narasimha Vara Prasad, Nanthi Bolan, Jörg Rinklebe, Rao Zepeng, Sabry M. Shaheen, Liheng Liu, Microbe-assisted phytoremediation of toxic elements in soils: Present knowledge and future prospects, 2024, 255, 00128252, 104854, 10.1016/j.earscirev.2024.104854 | |
4. | Cristina Calderón-Tapia, Edinson Medina-Barrera, Nelson Chuquin-Vasco, Jorge Vasco-Vasco, Juan Chuquin-Vasco, Sebastian Guerrero-Luzuriaga, Exploration of bacterial strains with bioremediation potential for mercury and cyanide from mine tailings in "San Carlos de las Minas, Ecuador", 2024, 11, 2372-0352, 381, 10.3934/environsci.2024019 | |
5. | Murat Huseyin UNSAL, Gytautas IGNATAVIČIUS, Roberta VALSKIENĖ, Vaidotas VALSKYS, 2023, Long-term heavy metal accumulation in sediment dust of schools in Vilnius: a case study, 978-609-476-342-7, 1, 10.3846/enviro.2023.901 | |
6. | Tri Prartono, Nyoman Metta Nyanakumara Natih, Mochamad Tri Hartanto, Agus Soleh Atmadipoera, Rifdina Afifah, Santi Susanti, Dewy Septiyanti Yolanda, Erwin Maulana, Lestari Lestari, Yulianto Suteja, Anna Ida Sunaryo Purwiyanto, Multi-metals analysis in sediment of the North Sumatra coast, Indonesia: The environmental status, 2023, 196, 0025326X, 115666, 10.1016/j.marpolbul.2023.115666 | |
7. | Dogo Lawrence Aleku, Olesya Lazareva, Thomas Pichler, Mercury in groundwater – Source, transport and remediation, 2024, 170, 08832927, 106060, 10.1016/j.apgeochem.2024.106060 | |
8. | Timothy Amangdam Anemana, Mohammed Buri, Collins Tay, Iodide- and electrochemical assisted removal of mercury by Cirsium arvense from gold tailings in the Amansie West District, Ghana , 2024, 26, 1522-6514, 2266, 10.1080/15226514.2024.2386302 | |
9. | Rakesh Pant, Pratyaksha Singh, Nirmal Patrick, Amit Gupta, 2024, Chapter 9, 978-3-031-48816-0, 201, 10.1007/978-3-031-48817-7_9 | |
10. | Robert Clough, Chris F. Harrington, Steve J. Hill, Yolanda Madrid, Julian F. Tyson, Atomic spectrometry update: review of advances in elemental speciation, 2023, 38, 0267-9477, 1339, 10.1039/D3JA90022C | |
11. | Sukanya Acharyya, Sumedha Saha, Arindam Ghosh, Soumya Majumder, Malay Bhattacharya, Mercury tolerance and bioremediation potential of mountain soil bacteria: Insights from Darjeeling, containing elevated levels of mercury, 2025, 960, 00489697, 178351, 10.1016/j.scitotenv.2024.178351 | |
12. | Esther Ugo Alum, The role of toxicology in climate change: Understanding the risks of novel environmental toxins, 2025, 11, 2765-8511, 10.1080/27658511.2025.2467485 |