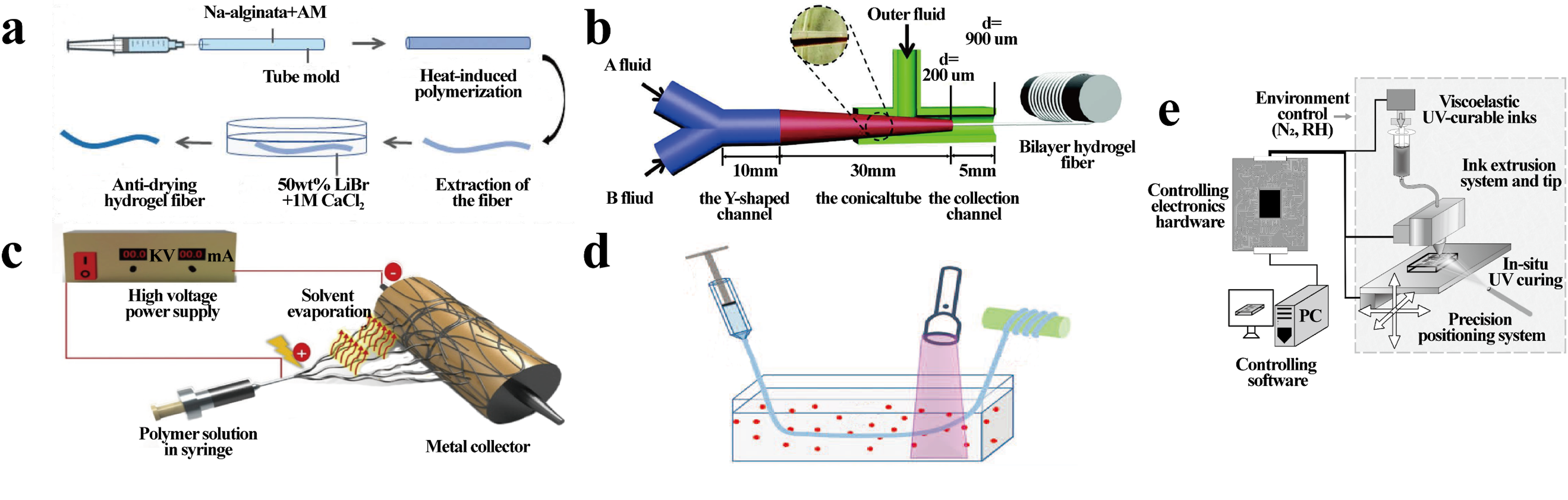
In the past few decades, many researchers have focused their research interests on nanocomposite hydrogel fibers (NHFs). These practitioners have developed and optimized techniques for preparing nanofiber membranes such as the template method, microfluidic spinning, electrospinning, wet spinning and three-dimensional printing (3D printing). NHFs have important applications in wearable monitoring, diagnosis and nursing due to their various excellent properties (such as high-water content, porous morphology, flexibility, braiding and rich active functional groups). In this paper, the latest progress of NHFs in pose monitoring, continuous monitoring of physiological indicators, diagnosis, wearables, nursing, drug delivery and dressings are reviewed. This paper also aims to review their key operational parameters, advantages and disadvantages of NHFs in the above fields, including sensitivity, working range and other special properties. Specifically, NHFs can be used for continuous monitoring of biological postures (such as gestures) or physiological indicators (such as blood sugar) in vitro and in vivo. NHFs also can be used for long-term monitoring of related indicators in the wearable field. NHFs can be used in tissue engineering and drug delivery. Finally, we look forward to the development prospects, challenges and opportunities of the next generation of NHFs. We confirm that the emergence of NHFs in the field of diagnosis and treatment has opened up a new vision for human health. Researchers have optimized the template method, microfluidic spinning, electrospinning, wet spinning and 3D printing.
Citation: Zhenguo Yu, Dong Wang, Zhentan Lu. Nanocomposite hydrogel fibers in the field of diagnosis and treatment[J]. AIMS Materials Science, 2023, 10(6): 1004-1033. doi: 10.3934/matersci.2023054
[1] | Mona Mohsen, Ehsan Gomaa, Nabila Ahmed Mazaid, Reem Mohammed . Synthesis and characterization of organic montmorillonite-polyvinyl alcohol-co-polyacrylic nanocomposite hydrogel for heavy metal uptake in water. AIMS Materials Science, 2017, 4(5): 1122-1139. doi: 10.3934/matersci.2017.5.1122 |
[2] | João Otávio Donizette Malafatti, Thamara Machado de Oliveira Ruellas, Camila Rodrigues Sciena, Elaine Cristina Paris . PLA/starch biodegradable fibers obtained by the electrospinning method for micronutrient mineral release. AIMS Materials Science, 2023, 10(2): 200-212. doi: 10.3934/matersci.2023011 |
[3] | Iketut Suarsana, Igpagus Suryawan, NPG Suardana, Suprapta Winaya, Rudy Soenoko, Budiarsa Suyasa, Wijaya Sunu, Made Rasta . Flexural strength of hybrid composite resin epoxy reinforced stinging nettle fiber with silane chemical treatment. AIMS Materials Science, 2021, 8(2): 185-199. doi: 10.3934/matersci.2021013 |
[4] | Elena Kossovich . Theoretical study of chitosan-graphene and other chitosan-based nanocomposites stability. AIMS Materials Science, 2017, 4(2): 317-327. doi: 10.3934/matersci.2017.2.317 |
[5] | Mercy Ogbonnaya, Abimbola P.I Popoola . Potential of mucilage-based hydrogel for passive cooling technology: Mucilage extraction techniques and elucidation of thermal, mechanical and physiochemical properties of mucilage-based hydrogel. AIMS Materials Science, 2023, 10(6): 1045-1076. doi: 10.3934/matersci.2023056 |
[6] | Vincenzo Guarino, Tania Caputo, Rosaria Altobelli, Luigi Ambrosio . Degradation properties and metabolic activity of alginate and chitosan polyelectrolytes for drug delivery and tissue engineering applications. AIMS Materials Science, 2015, 2(4): 497-502. doi: 10.3934/matersci.2015.4.497 |
[7] | Kyohei Fujita, Zhonggang Feng, Daisuke Sato, Tadashi Kosawada, Takao Nakamura, Yasuyuki Shiraishi, Mitsuo Umezu . Modulation of the mechanical properties of ventricular extracellular matrix hydrogels with a carbodiimide crosslinker and investigation of their cellular compatibility. AIMS Materials Science, 2018, 5(1): 54-74. doi: 10.3934/matersci.2018.1.54 |
[8] | Narayan Poudyal, Kinjal Gandha, Kevin Elkins, J. Ping Liu . Anisotropic SmCo5/FeCo core/shell nanocomposite chips prepared via electroless coating. AIMS Materials Science, 2015, 2(3): 294-302. doi: 10.3934/matersci.2015.3.294 |
[9] | Prashant Tripathi, Vivek Kumar Gupta, Anurag Dixit, Raghvendra Kumar Mishra, Satpal Sharma . Development and characterization of low cost jute, bagasse and glass fiber reinforced advanced hybrid epoxy composites. AIMS Materials Science, 2018, 5(2): 320-337. doi: 10.3934/matersci.2018.2.320 |
[10] | Mohsen Safaei, Mohammad Salmani Mobarakeh, Bahram Azizi, Ehsan Shoohanizad, Ling Shing Wong, Nafiseh Nikkerdar . Optimization of synthesis of cellulose/gum Arabic/Ag bionanocomposite for antibacterial applications. AIMS Materials Science, 2025, 12(2): 278-300. doi: 10.3934/matersci.2025015 |
In the past few decades, many researchers have focused their research interests on nanocomposite hydrogel fibers (NHFs). These practitioners have developed and optimized techniques for preparing nanofiber membranes such as the template method, microfluidic spinning, electrospinning, wet spinning and three-dimensional printing (3D printing). NHFs have important applications in wearable monitoring, diagnosis and nursing due to their various excellent properties (such as high-water content, porous morphology, flexibility, braiding and rich active functional groups). In this paper, the latest progress of NHFs in pose monitoring, continuous monitoring of physiological indicators, diagnosis, wearables, nursing, drug delivery and dressings are reviewed. This paper also aims to review their key operational parameters, advantages and disadvantages of NHFs in the above fields, including sensitivity, working range and other special properties. Specifically, NHFs can be used for continuous monitoring of biological postures (such as gestures) or physiological indicators (such as blood sugar) in vitro and in vivo. NHFs also can be used for long-term monitoring of related indicators in the wearable field. NHFs can be used in tissue engineering and drug delivery. Finally, we look forward to the development prospects, challenges and opportunities of the next generation of NHFs. We confirm that the emergence of NHFs in the field of diagnosis and treatment has opened up a new vision for human health. Researchers have optimized the template method, microfluidic spinning, electrospinning, wet spinning and 3D printing.
Hydrogel is a kind of three-dimensional cross-linked network polymer with water as the swelling agent [1,2]. It has the characteristics of good adjustability, good flexibility, easy degradation and biocompatibility [3,4,5,6]. However, there are also some shortcomings, such as easy cracking, freezing at low temperature, poor thermal stability and mechanical properties, and insufficient functionality [7,8,9,10,11]. One-dimensional nanocomposite hydrogel fibers (NHFs) have become a new favorite in the field of diagnosis/treatment research, and great progress has been made [12,13,14]. Compared with two-dimensional hydrogel films or three-dimensional hydrogel blocks, it has the advantages of excellent mechanical flexibility, good weaving, high orientation of polymer chains, light weight and small size of flexible electronic devices [15,16,17,18,19]. NHFs are a kind of nanocomposites with micro-three-dimensional cross-linked network structure and macro-one-dimensional fiber morphology [20,21]. It is composed of hydrogel and uniformly dispersed nano-scale materials with large specific surface area, rich active functional groups and rigid structure [15,22,23]. The hydrogel state makes NHFs have the characteristics of high-water content, porous morphology, intelligent stimulus response and high elasticity. The shape of the fiber makes NHFs have the characteristics of high specific surface area, flexibility, anisotropy, braiding and excellent mechanical properties. Nanoparticles endow NHFs with the characteristics of functional response and ability to regulate mechanical properties. It plays an important role in the fields of posture and physiological monitoring, nursing care, diagnosis and treatment, and tissue engineering [17,24,25,26,27].
The application of diagnosis and treatment technology has been closely related to human health. Diagnosis and treatment science and its principles play an important role in medicine [28,29]. Traditional diagnosis and treatment are independent. It cannot be effectively formed to implement the linkage mechanism. There are also disadvantages of time-consuming and expensive [30,31]. As a new type of medical technology, the integration of diagnosis and treatment has broad application prospects [32,33]. The integration of diagnosis and treatment realized by NHFs is a hot topic in today's research. In this context, NHFs can overcome several limitations [34,35,36]. In fact, the application of different NHFs in the field of human health is a promising way to solve various problems in this field. NHFs have been manufactured and applied in the field of diagnosis and treatment [37,38]. NHFs have important applications in wearable monitoring, diagnosis and nursing. Specifically, NHFs can be used for continuous monitoring of biological postures (such as gestures) or physiological indicators (such as blood sugar) in vitro and in vivo [14,39]. NHFs also can be used for long-term monitoring of related indicators in the wearable field. NHFs can be used in tissue engineering and drug delivery [40,41]. NHFs are endowed with unique and superior properties by their hydrogel state, fiber shape and functionalized nanoparticles. These properties of NHFs provide new means for the diagnosis and treatment of sensitive and reliable biological posture and physiological indicators.
Despite the great application prospects of NHFs, there are still some shortcomings. For example, the mechanical strength of NHFs is still low and the swelling is uncontrollable [15]. In addition, the swelling and insoluble crosslinking structure limits the deformation ability of the gel material and makes it difficult to form fibers [42,43]. If NHFs can overcome these shortcomings, they will be promising. To our knowledge, there is very little systematic discussion of NHFs for diagnostic medicine. This paper reviews the research of NHFs in monitoring, diagnosis, wearable devices and nursing treatment. The contributions and shortcomings of NHFs in these fields are introduced. Finally, we look forward to the next generation of NHFs and the remaining challenges, opportunities and strategies.
The preparation methods of NHFs are various. The molding of NHFs according to the order of crosslinking network and fiber structure molding can be divided into (a) hydrogel cross-linking after fiber forming, (b) fiber forming after hydrogel cross-linking and (c) hydrogel cross-linking during fiber formation [16,44,45,46,47,48,49,50]. The existence of crosslinking points ensures that the three-dimensional network structure inside the hydrogel does not easily decompose in the swelling state [51]. The formation of hydrogel crosslinking networks in fiber processing is more conducive to the continuous construction of hydrogel fibers [52]. In particular, researchers have optimized the template method, microfluidic spinning, electrospinning, wet spinning and three-dimensional printing (3D printing) so that they can use these technologies to obtain rapid cross-linking of pre-polymerized gel solutions [53,54,55,56,57]. The devices and technical routes for the preparation of NHFs under different technical backgrounds such as the template method, microfluidic spinning, electrospinning, wet spinning and 3D printing are illustrated in Figure 1.
The preparation of NHFs by the template method has been proved to be a simple, economical, efficient and intuitive method [21,58,59]. NHFs can be easily fabricated by flowing a hydrogel pre-crosslinking solution through the template or pouring it on the template. Jin's group had prepared the hydrogel fibers that were synthesized from acrylamide (AAm) and sodium alginate with a template method, which were then encapsulated in a layer of Ecoflex [60]. The hydrogel precursor solution was injected into the glass capillary with a syringe and then sealed to avoid the formation of bubbles during the heating process. During the injection process, the formation of bubbles should be minimized, otherwise the hydrogel fiber easily breaks during the stretching process. Then, the glass cap was put into the oven to realize the polymerization of the hydrogel precursor solution. The fiber was taken out of the glass capillary and immersed in 1 M CaCl2 for 30 min to obtain an ion-crosslinked hydrogel. At this point, a double-network hydrogel fiber with high toughness and stretchability was prepared (Figure 1a). The product has good proximity sensing capability, wide detection range (18 cm), short response/recovery time (90/90 ms) and high sensitivity (3.17% cm−1). It can accurately detect the proximity of human hands, including the number of fingers and different gestures. Yang's group used a medical syringe to inject the precursor into a glass cap, and then ultraviolet (UV) cross-linking was performed for 30 min. After curing, the glass cap was immersed in enough hydrofluoric acid solution for 20 min to obtain bare hydrogel fibers [61]. These can be stretched to more than 2000% of their initial length. Wang's group cooled the precursor of the hydrogel fiber in an ice bath, degassed it, and then injected it into the tube mold to prepare hydrogel fibers [62]. In addition, compared with silicon fibers, the implantation of hydrogel fibers significantly reduced the tissue response of implants/nerves. In summary, the results of this study prove the feasibility and superiority of this method. The use of hydrogel implants allows the development of novel therapeutic strategies for neuropsychiatric disorders.
General, microfluidic spun hydrogel fibers are considered promising candidates for loading and delivering biosensitive macromolecules or drugs because of their hydrogel properties [63]. However, the internal characteristics of macropores in the microfluidic spun hydrogel fiber and the rapid degradation and sedimentation of the fiber material hinder its application in a controlled environment to transport small molecules such as ampicillin [63]. Lee's group introduced a new method to produce improved antibiotic alginate fiber with high drug loading performance and delayed degradation characteristics [64]. His members used a low-polar isopropanol sheath flowing a microfluidic spinning system to dehydrate the alginate/ampicillin aqueous solution to prepare high-density fibers, improve drug loading efficiency and prolong the time of drug release. Ma's group prepared a continuous double-layer hydrogel fiber with one layer of calcium alginate hydrogel and a layer of linear poly (N-isopropylacrylamide)/calcium alginate/graphene oxide semi-interpenetrating hydrogel by microfluidic spinning [65].
The microfluidic spinning device consists of a Y-shaped channel, a conical tube, a collection channel and a winding device. The effect of the y-type flow channel is to enable the two fluids to form a stable parallel laminar flow after they meet. The use of conical tubes and outer channels ensures that the diameter of hydrogel fibers is more easily controlled. Finally, continuous hydrogel fibers are collected by the winding device (Figure 1b). The double-layer hydrogel fiber has a bend response to temperature and near-infrared light and a wide range of application value in the field of light drive. Khademhosseini's group fabricated photo-crosslinked micro-structured gelatin methacryloyl (GelMA) fibers with excellent cell responsiveness and processability using a microfluidic device engraved with grooves [66]. The microgrooves on the surface of these hydrogel fibers can effectively promote cellular encapsulation and adhesion. It can be used as a template to create fibrous tissue or tissue microstructure. These hydrogel fibers have good prospects in tissue engineering applications, such as scaffolds to promote cell-material interactions in blood vessels, muscles or skin.
After thirty years of rapid development, electrospinning has become one of the most important forming technologies of nanofibers [67,68]. The system for this type of spinning normally consists of three components: a supplier of high voltage, a needle connected to a syringe and a metal collector (Figure 1c). Chen's group used photo-crosslinked GelMA and electrospinning technology to construct a new type of aligned hydrogel microfiber scaffold for spinal cord regeneration [69]. The mechanical soft scaffold with high water content and high elasticity can be obtained by photo-crosslinked hydrogel technology. GelMA hydrogel electrospun fibers absorb more than six times the weight in water and have low Young's modulus. In vitro experiments have shown that photo-crosslinked hydrogel microfiber scaffolds can provide a good survival and metabolic environment for cells, promote cell proliferation, migration and differentiation, and directional extension of axons. The flexible biomimetic scaffold constructed by electrospun gel fibers can not only promote the migration of neural stem cells and induce their differentiation into nerve cells, but also inhibit the formation of glial scars and promote angiogenesis. Lee's group co-doped TiO2 in poly(vinyl alcohol) (PVA) solution and then added Glutaraldehyde (GA) to cross-link PVA [70]. The prepared polymer solution was sprayed from the tip of the syringe to the collector covered with aluminum foil using an electrospinning device to obtain cross-linked hydrogel fibers. Yuan's group dropped the aqueous phase prepared by poly(ethylene glycol) diacrylate (PEGDA) of the mixed nanoparticles into the oil phase dissolved with poly(ethylene glycol)-b-poly(L-lactide-co-caprolactone) (PELCL) or poly(L-lactic acid-co-glycolic acid) (PLGA) to obtain a suspension for electrospinning [71]. This method is expected to provide new ideas for the encapsulation and controlled release of bioactive drugs. This design provides a simple and efficient packaging technology for biomedical applications.
Wet spinning is an ancient spinning technology [72,73]. Wet spinning is the preferred method for chitosan and alginate fibers because it maintains a strong interchain hydroxyl force in their chemical structure [74,75,76,77]. Wallace's group successfully prepared coaxial biofibers with a wet spinning method by simultaneously injecting chitosan at a rate of 14 mL·h−1 and alginate solution at a rate of 25 mL·h−1 into a 2% (w/v) CaCl2 water coagulation bath through a port in a coaxial spinneret [72]. These biofibers have shown great potential as drug delivery platforms for advancing current drug delivery systems. Hygroscopic organogel fibers were designed via a wet-spinning strategy by Chen's group [78]. Members use the wet spinning strategy to pump the spinning solution into the coagulation solution using a syringe and a plastic tube for crosslinking. Wet spinning technology to manufacture hygroscopic photothermal fibers is shown in Figure 1d. Under 365 nm ultraviolet irradiation, the hydrogel precursor was injected into the coagulation bath to form a brittle and strongly fibrous hydrogel. Finally, the obtained hydrogel fibers were collected on an electric drum, and then completely replaced with a pure glycerol solvent to obtain a gel fiber. The obtained fibers are composed of hydrophilic polymer networks, hygroscopic solvents and photothermal/antibacterial silver nanoparticles, which have hygroscopicity, photothermal conversion ability and antibacterial properties. The product provides a new way for programmable and wearable moisture-absorbing material platforms and shows great potential in editable and wearable moisture-absorbing multifunctional devices.
3D printing is an emerging technology for NHF preparation [79,80]. Compared with other technologies, the method is simple and flexible. The 3D printer consists of a precision positioning system, an ink system and a software and hardware/software interface. In this method, in-situ UV curing can be used to localize the extrusion ink to prevent large-area diffusion of the ink (Figure 1e). Suo's group used polyacrylamide (PAM) polymer components as tackifiers to adjust the viscoelasticity and rheological behavior of the gel prepolymerization solution containing acrylamide (AM) monomers, so that the prepolymerization solution can still maintain a fibrous morphology when extruded into an acrylic acid substrate, and then formed a cross-linked network through later UV polymerization to prepare hydrogel fibers with excellent tensile properties [81]. Luo's group prepared 3D printed drug-loaded alginate/gelatin NHFs [82]. Through near-infrared irradiation, on-demand delivery of scaffold layer drugs was realized. Drug coating can reduce the free diffusion of drugs from the scaffold and achieve long-term sustained release of drugs. Wu's group fabricated a programmable deformable hydrogel fiber with high response speed and large output forces based on 3D printing technology [83]. Highly viscoelastic poly(acrylic acid-co-acrylamide) and poly(acrylic acid-co-N-isopropylacrylamide) solutions for their mixtures are printed into 3D structures through multiple nozzles, and then transferred to FeCl3 solution to form a solid carboxyl-Fe3+ coordination complex, which gelates the structure. The tensile fracture stress (2.38 MPa), fracture strain (802%) and elastic modulus (0.80 MPa) of the prepared gel fibers were significant. The excellent mechanical properties and strong interfacial adhesion of the gel fibers make the composite gel structure mechanically robust and produces a considerable output force under stimulation, which is more than 100 times the weight of the actuator.
The monitoring of the physical motion state and physiological indicators has always been a research hotspot. It is widely used in health care, rehabilitation treatment and man-machine interface. The rapid development of flexible electronic devices has aroused a research upsurge of flexible sensors based on hydrogels. Conductive polymer hydrogels are an important branch among them. They can convert mechanical deformation into electrical signal and organically combine a hydrophilic matrix with a conductive medium. They have attracted the attention of researchers due to their excellent properties such as high water-absorption, excellent tensile and electrochemical properties, good biocompatibility and high shape plasticity. Wu's group had prepared sodium alginate/polyaniline/graphene (SA/PANI/graphene) continuous hydrogel fiber by in-situ polymerization and wet spinning according to the device and process shown in Figure 2a [84]. The hydrogel precursor solution was squeezed into a Ca2+ coagulation bath. The precursor solution rapidly polymerized into PANI in the presence of APS. And then alginate rapidly formed ion-crosslinked fibers under the action of Ca2+. Hydrogel fibers were readily fabricated using a simple solvent displacement (Figure 2b). Hydrogel fiber could have been collected during the wet spinning, and the fibers could be easily knitted into a variety of textile, which shows the potential for wearable strain sensors [84]. It can be used as a wearable human motion monitoring strain sensor. The obtained hydrogel fiber has good flexibility, high water-absorption (11.37 g/g), appropriate electrical resistivity (220 Ω·m) and stable resistance changes at low (10%) and high (20% and 50%) strain (Figure 2c, d). It also demonstrated high sensitivity, flexibility and resilience when monitoring palm, elbow and knee movements (90% maintained after five cycles) (Figure 2e–g). This highly elastic and sensitive hydrogel makes it possible to prepare electromechanical sensors.
Traditional conductive polymer hydrogels are relatively fragile in the face of external stress and strain. Limb movement may reduce or damage the structure and function of the device. In order to improve the mechanical toughness, resilience, elasticity and flexibility of hydrogel devices, many studies have been carried out. Yun's group reported the design and manufacturing method of a core-sheath structure fiber with high tensile toughness and designed a novel and simple axial strain measurement technique [85]. In multiple cycles, fibers can be stretched to 700% axial strain. Wan's group developed a physically cross-linked poly(N-acryloyl-glycinamide-co-acrylamide)((poly (NAGA-co-Am) (PNA))) hydrogel fiber with excellent tensile properties, high conductivity and self-healing ability [16].The crosslinking mode inside the hydrogel fiber and the mechanism of self-healing ability (reversible sol-gel morphological transformation) are shown in Figure 3a. The hydrogel is physically crosslinked by hydrogen bonds between the amide groups on the side chain. Double hydrogen bonds can be formed between N-acryloyl-glycinamide (NAGA) segments and a single hydrogen bond is formed between the AAm segments. The optimal gel precursor should be able to transform into a viscous sol at an appropriate elevated temperature and undergo reversible gelation when cooled to room temperature. The fiber was 50% stretched and then released for, and the ΔR/R0 showed a stable and rapid responses to tensile stimulation during the whole cycle after 120 cycles of release after 50% stretching (Figure 3b). Hand gestures could be monitored and distinguished when fiber sensors were fixed on each of the five fingers. For example, "ok", "victory" and "claw" gestures show unique electronic signals. It has certain strain sensing ability and can be used to human movement monitoring (Figure 3c). The core-sheath fiber exhibits excellent anti-evaporation performance and water absorption performance while maintaining mechanical properties. It has broad application prospects in functional intelligent textiles and wearable electronic products.
However, hydrogel-based sensors still have some problems, such as poor water-retention and inability to overcome drying, which means they can only work at room temperature and relatively high humidity. Han's group prepared a poly 3, 4-dioxyethylene thiophene: polystyrene sulfonic acid@poly(vinyl alcohol) (PEDOT:PSS@PVA) hydrogel fiber strain sensor using dynamically cross-linked PVA hydrogel as a stretchable matrix and PEDOT:PSS as a conductive material [86]. The sensor has excellent sensitivity to small strains and anti-drying/anti-freezing properties. The minimum strain can reach 0.01%, the ultimate tensile strength can reach 13.76 MPa and the elongation at break can reach 519.9%. In addition, the sensor also showed excellent stability and frost resistance under extremely cold conditions at −60 ℃, and ΔR/R0 values did not change significantly after 1000 cycles at 10% repeated strain. The hydrogel fibers remain flexible after being placed in the atmosphere for 1 year. The above studies on NHFs in the field of biological posture and physiological indicator monitoring have confirmed the great potential of NHFs in human health monitoring.
Continuous monitoring of physiological indicators (for example blood glucose) is necessary for patients with chronic diseases [87,88]. It can guide patients to control the corresponding indicators and improve the quality of life of patients. It also reduces the frequency of sensor implantation/replacement, tissue damage in patients, and the time and money costs of doctors and patients.
Attaching the sensor to the hydrogel fibers increases its contact area with the underlying tissue, thereby reducing the mobility of the implant under the skin [89]. In order to reduce the inflammatory response, hydrogel fiber sensors need to have high biocompatibility [89]. Takeuchi's group made glucose-responsive fluorescent polyacrylamide (PAM) hydrogel fiber. The working route and appearance of the fluorescent hydrogel fiber for continuous monitoring of glucose are shown in Figure 4a, b. Fluorescent imaging showed that the glucose reactive monomer was immobilized in the hydrogel fibers. These hydrogel fibers can stay at the implant site for a long time and can continuously respond to changes in blood glucose concentration for up to 140 days [89,90]. As shown in Figure 4c–f, hydrogel fibers are easy to remove. Compared with PAM hydrogel fibers, the application of polyethylene glycol (PEG) can reduce macrophage adhesion to inflammatory proteins, such as albumin, fibrinogen and fibronectin, and effectively reduce the inflammation [89,91,92,93,94]. At the same time, the number of implanted fluorescent sensors can be easily controlled by cutting the optical fibers to the required length. This result implies the potential application prospect of NHFs sensors.
Further, Yun's group used the NHFs (AM, poly(ethylene glycol) diacrylate (PEGDA) and 3-AM phenylboronic acid (3-APBA) copolymer) to change their physical and optical properties under the action of glucose [44]. The design route of glucose-sensitive hydrogel fiber is shown in Figure 5. Calcium alginate-coated polyhydrogel fibers were prepared by in-situ polymerization in capillary mode. The glucose concentration was obtained by measuring the change of the transmitted light intensity through the NHFs. The reflection path of light in the fiber and the attenuation coefficient of light can be regulated, and the blood glucose concentration in the body can be monitored in real time [44].
Usually, it takes a long time for the NHFs to the balance of the glucose complex, which is not conducive to the rapid detection of glucose in the body. Based on the cutting-edge functional technology of silica and biocompatible hydrogel fibers, Butte's group developed a fiber probe for rapid preparation and continuous blood glucose monitoring [95]. The preparation process and detection principle of glucose hydrogel fiber for biocompatibility detection are shown in Figure 6. The prepared hydrogel sensor was detected at different glucose concentrations (0–50 mM). The sensor's response saturated with increasing glucose concentration. In the physiological range, the sensitivity and selectivity of the silica hydrogel fiber probe to glucose was higher than that of lactic acid. The readout method is simple, practical, efficient and low cost. Optical data can be recorded by smartphone or optical power meters without data processing or expensive equipment. Therefore, it has a great application prospect in clinical trials.
Nowadays, researchers focus on wearable electronics with biological posture and physiological index monitoring. Benefiting from the rapid development in sensor technology, high-performance wearable sensors have been applied in human health monitoring. The applications of wearable sensors are divided into biophysical tracking, biochemical monitoring and real-time data detection [80,96,97,98,99,100]. Thanks to the intelligent stimulus response, high elasticity, flexibility, braiding and other characteristics of NHFs, hydrogel fibers can be easily woven into a variety of complex geometric shapes or integral textiles, and they have great potential for being woven into smart devices [101].
An important challenge for hydrogel fibers spinning is the dynamic control of spinning: spinning is a dynamic process, solid fiber segments are formed instantaneously under uniaxial tension, and the molecules are anisotropic [94,102,103]. It is difficult to achieve continuous and stable spinning. At present, most of the reported preparation methods of hydrogel fibers are similar to slow capillary kinetic in-situ polymerization [104]. It can only produce hydrogel fibers with limited fiber length and cannot be continuously produced on a large scale.
Recent advances in pre-gel technology seem to be able to overcome the challenges of spinning continuous fibers [105,106]. The extrusion process inspired by the characteristics of Chinese "Hele noodle" has been shown to be used to rapidly manufacture hydrogel fibers using covalently cross-linked dispersions according to the device and process shown in Figure 7a [47]. The prepolymer solution was filled into a syringe without an adaptor part and exposed to 365 nm UV light for photo-crosslinking. By squeezing the hydrogel through a scientific sieve, thousands of hydrogel surfaces are made in seconds. Inspired by this, Zhu's group prepared NHFs with weakly gelled nanoparticles/oligomers (cellulose nanocrystals/poly(ethylene glycol) diacrylate (CNC/PEGDA)) dispersions based on a novel template-free dynamic cross-linked spinning method [104]. The design route is shown in Figure 7b. Therefore, a filament-shaped CNC/PEGDA fluid phase was formed after being extruded into water due to the viscoelasticity of CNC/PEGDA dispersion. Instantaneous photopolymerization was triggered by UV irradiation of the liquid phase, and the liquid phase wasrapidly solidified into a hydrogel filament. Through dynamic extrusion, the filament-shaped CNC/PEGDA hydrogel could be collected continuously out of water by a roller. Compared with PEGDA, the addition amount of CNC was relatively small (0.96 wt%), however, the fiber fracture strength increased from 5.5 to 6.7 MPa. When CNC content is high (1.27 wt%), the cross-linked density of hydrogel fibers increases with the addition of CNC (Figure 7c–f). It limits the deformation of hydrogel fibers under tensile action and can be better used for continuous spinning of hydrogel fibers. Similarly, Zhou's group prepared CNC/PVA NHFs with the idea shown in Figure 8a. The hydrogel fibers were rapidly covalently cross-linked under UV irradiation and subsequently reacted with strong hydrogen bonds derived from tannic acid (TA) [107]. The prepared hydrogel fiber has flexibility and mechanical stability. The tensile strength is 7.3 MPa and the elongation at break is 123.8% (Figure 8b–g). Compared with PVA-glycidyl methacrylate hydrogel, the multi-cross-linked skeleton structure makes the hydrogels have excellent tensile strength and high-water content. The prepared hydrogel fibers can be easily woven into a variety of complex geometries or whole textiles, indicating that they have great potential for weaving smart devices.
Furthermore, Fei's group used a double-network (DN) hydrogel precursor by wet-spinning to obtain fiber filaments with controllable elongation [108]. In order to prevent the covalent cross-linking of the precursor before spinning, the pH of the polyacrylamide-co-diacetone acrylamide-5/ iota-carrageenan (PAD-5/IC) hydrogel precursor was adjusted to 1.0 and the syringe temperature was kept at 70 ℃ to prevent covalent crosslinking (Figure 9a). The wet-spinning process is demonstrated in Figure 9b. The strength and toughness of the obtained fibers were 1.35 MPa and 1.22 MJ/m3, respectively. The hydrogel fiber and its bulk sample have efficient self-healing properties (Figure 9c–f). By simultaneously repairing covalent networks and supramolecular networks under acidic and heated conditions, the fibers achieved rapid and almost complete repair with a repair efficiency of 96%. The hydrogel fibers with self-healing properties and super-toughness are used as shape memory materials. Facts show that NHFs have potential in smart fabrics.
Power supply and transmission is another important challenge for the application of hydrogel fiber electronic devices in the wearable field. Self-power is considered to be a development trend for electronic equipment in the field of wearable applications [109]. The moisture and softness of hydrogels make them an ideal choice for electrolytes in flexible energy storage devices such as supercapacitors and rechargeable batteries.
Stretchable all-solid-state supercapacitors have high power density, fast charging/discharging speed and excellent mechanical properties [110,111,112,113,114]. It is considered to be one of the most promising power sources in scalable electronic devices, and it is crucial to the development of smart electronic textiles. All-solid-state supercapacitors can obtain excellent tensile properties by twisting the hydrogel electrolyte-coated electrode material into a fibrous shape [113,115,116,117]. Conductive polymers are often used as active electrode materials, such as PANI, polypyrrole, polythiophene and poly (3, 4-ethyloxythiophene) [118]. They are ideal electroactive materials for constructing all-solid-state supercapacitors due to their high theoretical capacity, excellent electrochemical activity, light weight and high flexibility. Converting thermal energy and mechanical energy from the human body as well as solar energy into electricity energy is considered to be a feasible energy source [119,120]. The slow response speed and poor mechanical properties of electrically responsive hydrogels limit their practical application [121].
In order to solve these problems, researchers have done a lot of work and made great progress. Conducting nanomaterials such as graphene and carbon nanotubes are usually introduced to form nanocomposite hydrogels, which improve the electrical response rate and enhance the mechanical properties. Ma's group prepared Graphite Oxide (GO)/PAM/sodium alginate hydrogel fibers with high tensile and fast electrical response [121]. The results show that the prepared hydrogel fibers have strong tensile properties by adjusting the proportion and dosage of nano-composites, such as GO and N, N-methylene-bis-acrylamide. Compared with hydrogel rods, micro-scale diameter hydrogel fibers have greater advantages, such as faster expansion rate and electrical reaction rate. The thinner the hydrogel fiber, the faster the electrical reaction.
The invention of thin hydrogel fibers has enabled the application of hydrogel fibers with high tensile strength and fast electrical response in the field of artificial muscle actuators [121]. Natural biological hydrogels, such as muscle or articular cartilage, often have high strength and toughness by orienting hydrophilic network structures. Therefore, anisotropic hydrogel fibers with highly oriented structures have attracted more and more attention. Xu's group prepared highly conductive PEDOT:PSS hydrogel fibers post-treated with organic solvents [118]. The solvent post-treatment leads to the peeling of the insulating PSS shell and the exposure of the conductive PEDOT core, and the conductivity increases up to 172.5 S·cm−1. Yu's group prepared PANI nanocomposite gels doped with GO according to the process shown in Figure 10a. The team prepared polyaniline/reduced graphene oxide nanocomposite gel fibers at 180 ℃ using ultrasound-dissolved gel and ascorbic acid using a capillary as a template [53,122]. The gel fiber can be reshaped into a gel-state supercapacitor with a spring-like structure including elasticity and shape memory. It is possible to manufacture integrated energy storage yarns to power wearable and portable electronic devices.
With the development of stretchable electronic devices, the ability of stretchable energy storage devices to maintain stable operation under great mechanical strain is becoming more and more important. Ma's group prepared conductive hydrogel fibers with ordered polymer chains using sodium polyacrylate (PAAS) by mimicking the spider silk drawing process with the idea shown in Figure 10b [123]. This group further prepared core-sheath waterproof polymethyl acrylate-sodium polyacrylate (PMA-PAAS) hydrogel (MAPAH) fibers, which were coated with a thin layer of polymethyl acrylate on PAAS hydrogel (PAH).
The team proposed a mechanism to explain the unique mechanical behavior of MAPAH fibers (Figure 10c). Since MAPAH fibers contain a large amount of water, water as solvent and plasticizer, the amorphous domain can be forced to form ordered arrangement during stretching, thereby providing a high stretchability and high strength. After the release of stretched fiber, the temporarily arranged PAAS chains will be relaxed, and the entropy favorable random image and orientation can be quickly restored with the help of water in the fibers. It should be noted that due to the coexistence and reversible transformation of crystalline and amorphous domains during the spinning and gelation of hydrogel fibers, the waterproof MAPAH fiber prepared by Ma's group has high tensile strength (5.6 ± 0.6 MPa) and tensile properties (elongation at break of 1180 ± 100%), rapid resilience under large tensile strain (30 s), high electrical conductivity (200 S·cm−1) and excellent anti-freezing performance (at −35 ℃, the tensile and electrical conductivity of MAPAH fibers remain unchanged) [123]. As a high-performance and low-cost stretchable conductive fiber, multifunctional MAPAH fiber will provide guidance for the design of next-generation stretchable electronic devices based on textiles. Zhang's group prepared a gel prepolymer solution by in-situ polymerization in a tubular mold and demolded it at room temperature according to the process shown in Figure 11a–d [46,124]. The formation of PEDOT:PSS hydrogel at room temperature is initially attributed to the physical crosslinking between PEDOT+ polymer chains, which is achieved by the addition of DBSA. These PEDOT:PSS hydrogels spontaneously formed after the syringe injected the PEDOT:PSS suspension into the desired position, without the need of any additional treatment (Figure 11e–l). The group designed an injectable conductive hydrogel using the room temperature gelation ability of PEDOT:PSS suspensions. It is expected to play an important role in organic bioelectronic devices, including organic electrochemical transistors (OECTs), implantable medical devices, wearable devices, electronic skin, soft robots and soft neural electronics [121,124,125,126,127,128].
Hydrogel fibers have good tissue matching performance in structure and biological function, which has attracted the wide attention of researchers. They have a three-dimensional network structure with a high specific surface area [129]. Therefore, they can simulate the structure and properties of natural extracellular matrix, providing more sites and a suitable substrate environment for cell adhesion and growth [69,130]. At the same time, hydrogels have the characteristics of high water content, high porosity, high elasticity, high response to environmental stimulation and high swelling, and have a high degree of compatibility with the structure and behavior of human skin tissue [39,131,132]. In addition, its excellent hydrophilicity enables it to maintain the water balance of the skin wound surface [132,133]. By mimicking the complex structure and function of natural skin tissue, hydrogel fibers promote wound healing and provide appropriate mechanical properties and elasticity for wound healing treatment [134]. This makes them suitable candidates for skin regeneration. Hydrogel fibers have broad application prospects in the field of tissue engineering, drug delivery and other nursing treatment [41,135].
Researchers can incorporate different therapeutic drugs (such as antibacterial agents, silver ions, N-acetaminophen glucose, etc.) into hydrogel fibers to promote wound healing [40,129,130,136]. Hydrogel fiber excipients simulate the structure and properties of the natural extracellular matrix, providing convenience for delivery of therapeutic drugs to cell [40]. Rafailovich's group prepared hyaluronic acid hydrogel fibers [137]. They added polyethylene oxide in the spinning solution as a viscosity regulator to promote the formation of fibers. Polyethylene oxide was selectively removed with water after the electrospinning process. This prepared three-dimensional hydrogel fiber scaffold can promote the vascularization of endothelial cells [138]. Cui's group prepared calcium phosphate nanoparticles by the emulsion method [139]. They bonded gelatin methylacrylyl by electrospinning fibers to construct hybrid hydrogel fibers with protein domains similar to those of natural ECMs. Figure 12a shows the electrospun fibers have osteogenic ability in vitro. After 14 and 21 days of co-cultures, the mineralization behavior was time-dependent. As shown in Figure 12b, with the increase of time, more significant osteoblast metabolic activity can be found in cells treated with the CaPs loaded GelMA hydrogel fibers, which was manifested as deeper color and larger staining area. Quantitative results about osteogenesis in vitro were presented in Figure 12c–e. The use of biomimetic organic-inorganic hybrid hydrogel electrospun periosteum to accelerate the bone regeneration is shown in Figure 12f. It shows potential angiogenesis and bone formation. Liu's group prepared self-assembled hydrogel fibers containing human placental aqueous extract by using the wound healing effect and modified N-acetaminophen glucose [40]. It not only has antibacterial properties, but also can promote angiogenesis and treat chronic ulcerative wounds. Luo's group used co-axial injection and 3D printing technology to prepare core-shell fibers and scaffolds with near infrared trigger administration for local cancer treatment and combination with surgery [140]. In the core-shell fiber, the PDA/alginate shell with good photothermal effect induced the gelation of the drug-loaded core hydrogel under near-infrared laser irradiation. Then, the drug was released on demand from the open end of the fiber, effectively killing breast cancer cells and inhibiting tumor growth [140].
A wound that cannot achieve anatomical and functional integrity through a normal, orderly and timely repair process is called a chronic wound. Chronic wound is one of the worst complications of diabetes and accelerating angiogenesis in the early stage after injury is essential for wound healing [141]. Liu's group has developed a drug-loaded gel fiber scaffold, as shown in Figure 12g, h, to promote rapid blood vessel formation and wound healing [142]. The release period of the biological factor desferrioxamine can reach 3 days, and the hydrogel fiber scaffold could degrade within 14 days. Compared with the traditional biological scaffold, the degradation of scaffold leaves enough space for cell proliferation and angiogenesis. Neovascularization is significantly increased and the wound healing is significantly accelerated.
In order to overcome the shortcomings of low drug loading effect, fast drug release speed and instability of ordinary fiber platform, a lot of research has been carried out. Cui's group developed electrospun NHFs according to the process shown in Figure 13a, b [143]. Using nanoparticles (1–1000 nm) solution (sol) and crosslinking agent, gelation occurs to produce nanoparticles with a stable internal network structure. After the gel is formed, a fiber membrane containing uniformly distributed nanogel particles and carrying water-soluble drugs is fabricated.
It can load the water-soluble drug chloroquine, thereby achieving more stable loading and longer water-soluble drug release. In addition to being loaded inside and outside the nanogel particles, chloroquine is also distributed in the gel fibers and released for up to 40 days. Khademhosseini's group constructed an electrospun three-dimensional fiber scaffold using an optically cross-linked hydrogel based on gelatin methacrylate [144]. The optimized hydrogel fiber scaffold is soft and flexible enough to support cell adhesion, proliferation and migration throughout the scaffold. Neibert's group prepared an alginate fiber loaded with silver nanoparticles [130]. This method provides a convenient platform for incorporating silver nanoparticles of a specific concentration into alginate fibers while maintaining the mechanical and expansion properties of alginate fibers. The fiber has been well-applied in a series of cell experiments in vitro and experimental animal (mouse) wound models in vivo.
Hydrogels have become the most promising dressings due to their excellent biocompatibility, extracellular matrix simulation structure and drug loading capacity [145,146,147]. However, the existing hydrogel dressings have problems such as low structural strength, limited permeability, poor environmental adaptability, potential drug resistance and limited drug options, which greatly limit their therapeutic efficacy [148,149,150,151,152].
In order to improve the structural strength of hydrogel dressing, many studies have been carried out. Fu prepared anisotropic alginate fiber nanowires containing hydroxyapatite based on a stress-induced method according to the process shown in Figure 14a [101]. Alginate solution forms hydrogels immediately upon contact with Ca2+, and fibers were formed after the glass substrate was completely by excessive hydrogel. The scanning electron microscopy (SEM) images are shown in Figure 14b–d. The surface morphology and the internal structure composed of compact dense nanofibrils indicate that it has good flexibility and structural integrity.
The scheme improves its mechanical properties. The maximum Young's modulus of the material is 4300 MPa and the tensile strength is 153.8 MPa. A multi-scale strengthening mechanism is proposed: The longitudinal arrangement of nanofibers is highly ordered and dense at the micro scale (Figure 14e, f) [101]. Structures of the acidic hydrogel are stabilized due to intermolecular hydrogen bonds, while the structure of the Ca-alginate hydrogel is stable due to ionic crosslinking. The addition of HAP nanowires does not destroy the anisotropic microstructure of the alginate fiber. Figure 14g shows the longitudinal cross-section image of the sample. At the nanoscale, two alginate gels (acidic hydrogel with stable intermolecular hydrogen bonds and calcium alginate saline gels with ionic cross-linking) with different mechanical behaviors coexist [101]. It provides a basis for further understanding the formation principles and mechanical properties of alginate biomimetic fibers. This bionic mechanical force-directed manufacturing strategy has guiding significance for the design of high-performance materials with anisotropic structures. Zhu's group used Al2(SO4)3 aqueous solution as a coagulant to prepare carboxymethyl cellulose hydrogel fibers by the solution spinning method [153]. The cross section of carboxymethyl cellulose hydrogel fiber is smooth and uniform, without cracking and peeling. When CMC substitution degree is 0.4, the tensile strength of hydrogel fiber is similar to that of cotton fiber, and the elongation at break is obviously better than that of cotton fiber. In addition, the hydrogel fiber has excellent moisture absorption performance. In equilibrium state, the distilled water absorption rate of hydrogel fiber is as high as 712%, which is very suitable for biomedical applications such as wound dressing.
Breathable hydrogel fiber excipients contribute to wound healing [154]. In order to improve the air permeability of hydrogel fiber excipients, Pan's group prepared a new type of gelatin glycerin hydrogel fiber based on Hofmeister effect wet spinning [152]. A schematic diagram of the wet spinning process and molecular evolution of glyhydrogel fibers is shown in Figure 15a–d. It showed the structural evolution of the preparation stage, including (a) spinning solution, (b) hydrogel fiber, (c) crosslinked hydrogel fiber and (d) crosslinked glyhydrogel fiber. Due to their unique knitting structure, textile dressings have excellent air permeability (1800 times higher than that of commercially available 3M dressings) and tensile strength (535.51 ± 38.66%). In addition, textile dressings can withstand extreme temperatures of −80 ℃, showing the potential for application in sub-zero environments. The introduction of glycerin makes textile dressings have significant antibacterial properties and expands the range of drug loading options. The novel gelatin glycerin hydrogel fabric dressing has a good effect on the healing of infected wounds and can completely close the skin of rats within 14 days (Figure 15e–g). These are the functions that traditional hydrogel dressings cannot achieve, which provide a new way for development of hydrogel dressings.
It can be concluded that NHFs have the characteristics of hydrogels, fibers and nanoparticles. They have the advantages of high-water content, porous morphology, intelligent stimulus response, high elasticity, rich active functional groups, flexibility, braiding, excellent mechanical properties, rigid structure, functional response and regulation of mechanical properties. The advantages of some studies are introduced, such as: (a) The construction of the core-sheath structure enables NHFs to obtain excellent anti-evaporation performance and water absorption performance while maintaining mechanical properties. (b) The introduction of conductive nanomaterials (graphene or carbon nanotubes) improves the electrical response rate of NHFs. (c) The construction of three-dimensional NHF fiber scaffolding is used to promote wound healing. (d) A multi-scale strengthening mechanism is introduced to enhance the mechanical properties of NHFs. It plays an important role in the monitoring of biological posture and physiological indicators, continuous monitoring of biological physiological indicators, wearable electronic devices for diagnosis, self-powered wearable electronic devices, drug-loaded NHFs and NHF dressings.
However, the existing hydrogel fiber materials have some defects. In order to realize the practicability of NHFs, much work must be done to overcome these defects. (a) Mechanical properties: Stimuli-responsive polymer networks and nano-materials can be introduced into NHFs to make them multifunctional with better mechanical properties. (b) Response speed: The response time of conductive hydrogel fiber sensors to external stimuli ranges from tens of seconds to min. There is still a gap between this response speed and the clinical demands for rapid detection. The response speed of the device needs to be improved. The application of highly elastic soft substrates on the sheath makes the sensors have excellent resilience and super-stretchability, which may become one of the future research directions. (c) Cycle stability: The reliability/stability of long-term operation still needs to be strengthened. Solid polymer-based ionic conductors have high stretchability, excellent conductivity and versatility. (d) Continuous production: Large-scale industrial production is the prerequisite for the application of NHFs, but it still faces challenges. The industrial application of NHFs depends on interdisciplinary integration and the joint efforts of the whole industry.
The authors declare they have not used Artificial Intelligence (AI) tools in the creation of this article.
All authors contributed to the editing of the manuscript. This work was supported by National Natural Science Foundation of China (52273060), National key research and development program (2022YFB3805803), Key Program of Department of Education of Hubei Province (NO.
D20221703). The authors thank the "Wuhan Engineering Technology Research Center for Advanced Fibers" for providing partial support for material processing.
The authors declare no conflict of interest.
[1] |
Azeem MK, Islam A, Khan RU, et al. (2023) Eco-friendly three-dimensional hydrogels for sustainable agricultural applications: Current and future scenarios. Polym Adv Technol 34: 3046–3062. https://doi.org/10.1002/pat.6122 doi: 10.1002/pat.6122
![]() |
[2] |
Hou Y, Ma S, Hao J, et al. (2022) Construction and ion transport-related applications of the hydrogel-based membrane with 3D nanochannels. Polymers 14: 4037. https://doi.org/10.3390/polym14194037 doi: 10.3390/polym14194037
![]() |
[3] |
Matole V, Digge P (2022) A brief review on hydrogel. RJTCS 13: 99–100. https://doi.org/10.52711/2321-5844.2022.00016 doi: 10.52711/2321-5844.2022.00016
![]() |
[4] |
Ghandforoushan P, Alehosseini M, Golafshan N, et al. (2023) Injectable hydrogels for cartilage and bone tissue regeneration: A review. Int J Biol Macromol 246: 125674. https://doi.org/10.1016/j.ijbiomac.2023.125674 doi: 10.1016/j.ijbiomac.2023.125674
![]() |
[5] |
Tang Y, Wang H, Liu S, et al. (2022) A review of protein hydrogels: Protein assembly mechanisms, properties, and biological applications. Colloid Surface B 220: 112973. https://doi.org/10.1016/j.colsurfb.2022.112973 doi: 10.1016/j.colsurfb.2022.112973
![]() |
[6] |
Almajidi YQ, Gupta J, Sheri FS, et al. (2023) Advances in chitosan-based hydrogels for pharmaceutical and biomedical applications: A comprehensive review. Int J Biol Macromol 253: 127278. https://doi.org/10.1016/j.ijbiomac.2023.127278 doi: 10.1016/j.ijbiomac.2023.127278
![]() |
[7] |
Mahfoudhi N, Boufi S (2016) Poly (acrylic acid-co-acrylamide)/cellulose nanofibrils nanocomposite hydrogels: Effects of CNFs content on the hydrogel properties. Cellulose 23: 3691–3701. https://doi.org/10.1007/s10570-016-1074-z doi: 10.1007/s10570-016-1074-z
![]() |
[8] |
Chen F, Zhou D, Wang J, et al. (2018) Rational fabrication of anti-freezing, non-drying tough organohydrogels by one-pot solvent displacement. Angew Chem Int Ed 57: 6568–6571. https://doi.org/10.1002/anie.201803366 doi: 10.1002/anie.201803366
![]() |
[9] |
Sun K, Cui S, Gao X, et al. (2023) Graphene oxide assisted triple network hydrogel electrolyte with high mechanical and temperature stability for self-healing supercapacitor. J Energy Storage 61: 106658. https://doi.org/10.1016/j.est.2023.106658 doi: 10.1016/j.est.2023.106658
![]() |
[10] |
Bin Asghar Abbasi B, Gigliotti M, Aloko S, et al. (2023) Designing strong, fast, high-performance hydrogel actuators. Chem Commun 59: 7141–7150. https://doi.org/10.1039/D3CC01545A doi: 10.1039/D3CC01545A
![]() |
[11] |
Wei Z, Gerecht S (2018) A self-healing hydrogel as an injectable instructive carrier for cellular morphogenesis. Biomaterials 185: 86–96. https://doi.org/10.1016/j.biomaterials.2018.09.003 doi: 10.1016/j.biomaterials.2018.09.003
![]() |
[12] |
Ran C, Wang J, He Y, et al. (2022) Recent advances in bioinspired hydrogels with environment-responsive characteristics for biomedical applications. Macromol Biosci 22: 2100474. https://doi.org/10.1002/mabi.202100474 doi: 10.1002/mabi.202100474
![]() |
[13] |
Sokolov P, Samokhvalov P, Sukhanova A, et al. (2023) Biosensors based on inorganic composite fluorescent hydrogels. Nanomaterials 13: 1748. https://doi.org/10.3390/nano13111748 doi: 10.3390/nano13111748
![]() |
[14] |
Su M, Ruan L, Dong X, et al. (2022) Current state of knowledge on intelligent-response biological and other macromolecular hydrogels in biomedical engineering: A review. Int J Biol Macromol 227: 472–492. https://doi.org/10.1016/j.ijbiomac.2022.12.148 doi: 10.1016/j.ijbiomac.2022.12.148
![]() |
[15] |
Li W, Liu J, Wei J, et al. (2023) Recent progress of conductive hydrogel fibers for flexible electronics: Fabrications, applications, and perspectives. Adv Funct Mater 33: 2213485. https://doi.org/10.1002/adfm.202213485 doi: 10.1002/adfm.202213485
![]() |
[16] |
Shuai L, Guo ZH, Zhang P, et al. (2020) Stretchable, self-healing, conductive hydrogel fibers for strain sensing and triboelectric energy-harvesting smart textiles. Nano Energy 78: 105389. https://doi.org/10.1016/j.nanoen.2020.105389 doi: 10.1016/j.nanoen.2020.105389
![]() |
[17] |
Wang XQ, Chan KH, Lu W, et al. (2022) Macromolecule conformational shaping for extreme mechanical programming of polymorphic hydrogel fibers. Nat Commun 13: 3369. https://doi.org/10.1038/s41467-022-31047-3 doi: 10.1038/s41467-022-31047-3
![]() |
[18] |
Štular D, Kruse M, Župunski V, et al. (2019) Smart stimuli-responsive polylactic acid-hydrogel fibers produced via electrospinning. Fiber Polym 20: 1857–1868. https://doi.org/10.1007/s12221-019-9157-8 doi: 10.1007/s12221-019-9157-8
![]() |
[19] |
Kim K, Choi JH, Shin M (2021) Mechanical stabilization of alginate hydrogel fiber and 3D constructs by mussel-inspired catechol modification. Polymers 13: 892. https://doi.org/10.3390/polym13060892 doi: 10.3390/polym13060892
![]() |
[20] |
Luo Y, Shoichet MS (2004) A photolabile hydrogel for guided three-dimensional cell growth and migration. Nat Mater 3: 249–253. https://doi.org/10.1038/nmat1092 doi: 10.1038/nmat1092
![]() |
[21] |
Du J, Ma Q, Wang B, et al. (2023) Hydrogel fibers for wearable sensors and soft actuators. iScience 26: 106796. https://doi.org/10.1016/j.isci.2023.106796 doi: 10.1016/j.isci.2023.106796
![]() |
[22] |
Sun W, Zhao X, Webb E, et al. (2023) Advances in metal-organic framework-based hydrogel materials: Preparation, properties and applications. J Mater Chem A 11: 2092–2127. https://doi.org/10.1039/D2TA08841J doi: 10.1039/D2TA08841J
![]() |
[23] |
Norahan MH, Pedroza-González SC, Sánchez-Salazar MG, et al. (2023) Structural and biological engineering of 3D hydrogels for wound healing. Bioact Mater 24: 197–235. https://doi.org/10.1016/j.bioactmat.2022.11.019 doi: 10.1016/j.bioactmat.2022.11.019
![]() |
[24] |
Li M, Chen X, Li X, et al. (2021) Wearable and robust polyimide hydrogel fiber textiles for strain sensors. ACS Appl Mater Interfaces 13: 43323–43332. https://doi.org/10.1021/acsami.1c10055 doi: 10.1021/acsami.1c10055
![]() |
[25] |
Yao B, Wang H, Zhou Q, et al. (2017) Ultrahigh-conductivity polymer hydrogels with arbitrary structures. Adv Mater 29: 1700974. https://doi.org/10.1002/adma.201700974 doi: 10.1002/adma.201700974
![]() |
[26] |
Rezapour Sarabi M, Jiang N, Ozturk E, et al. (2021) Biomedical optical fibers. Lab Chip 21: 627–640. https://doi.org/10.1039/D0LC01155J doi: 10.1039/D0LC01155J
![]() |
[27] |
Zaszczyńska A, Niemczyk-Soczynska B, Sajkiewicz P (2022) A comprehensive review of electrospun fibers, 3D-printed scaffolds, and hydrogels for cancer therapies. Polymers 14: 5278. https://doi.org/10.3390/polym14235278 doi: 10.3390/polym14235278
![]() |
[28] |
Mahaman YAR, Embaye KS, Huang F, et al. (2022) Biomarkers used in Alzheimer's disease diagnosis, treatment, and prevention. Ageing Res Rev 74: 101544. https://doi.org/10.1016/j.arr.2021.101544 doi: 10.1016/j.arr.2021.101544
![]() |
[29] |
Chen YH, Lou JG, Yang ZH, et al. (2022) Diagnosis, treatment, and prevention of severe acute hepatitis of unknown etiology in children. World J Pediatr 18: 538–544. https://doi.org/10.1007/s12519-022-00581-x doi: 10.1007/s12519-022-00581-x
![]() |
[30] |
Kher C, Kumar S (2022) The application of nanotechnology and nanomaterials in cancer diagnosis and treatment: A review. Cureus 14: 29059. https://doi.org/10.7759/cureus.29059 doi: 10.7759/cureus.29059
![]() |
[31] |
Xu W, Qing X, Liu S, et al. (2022) Hollow mesoporous manganese oxides: Application in cancer diagnosis and therapy. Small 18: 2106511. https://doi.org/10.1002/smll.202106511 doi: 10.1002/smll.202106511
![]() |
[32] |
Song J, Zhang Y, Chan SY, et al. (2021) Hydrogel-based flexible materials for diabetes diagnosis, treatment, and management. Npj Flex Electron 5: 26. https://doi.org/10.1038/s41528-021-00122-y doi: 10.1038/s41528-021-00122-y
![]() |
[33] |
Li Q, Cao Y, Wang P (2022) Recent advances in hydrogels for the diagnosis and treatment of dry eye disease. Gels 8: 816. https://doi.org/10.3390/gels8120816 doi: 10.3390/gels8120816
![]() |
[34] |
Zhu JQ, Wu H, Li ZL, et al. (2022) Responsive hydrogels based on triggered click reactions for liver cancer. Adv Mater 34: 2201651. https://doi.org/10.1002/adma.202201651 doi: 10.1002/adma.202201651
![]() |
[35] |
Ma Y, Huang J, Song S, et al. (2016) Cancer-targeted nanotheranostics: Recent advances and perspectives. Small 12: 4936–4954. https://doi.org/10.1002/smll.201600635 doi: 10.1002/smll.201600635
![]() |
[36] |
Tehrany PM, Rahmanian P, Rezaee A, et al. (2023) Multifunctional and theranostic hydrogels for wound healing acceleration: An emphasis on diabetic-related chronic wounds. Environ Res 238: 117087. https://doi.org/10.1016/j.envres.2023.117087 doi: 10.1016/j.envres.2023.117087
![]() |
[37] |
Gupta BD, Pathak A, Shrivastav AM (2022) Optical biomedical diagnostics using lab-on-fiber technology: A review. Photonics 9: 86. https://doi.org/10.3390/photonics9020086 doi: 10.3390/photonics9020086
![]() |
[38] |
Zhou C, Wu T, Xie X, et al. (2022) Advances and challenges in conductive hydrogels: From properties to applications. Eur Polym J 177: 111454. https://doi.org/10.1016/j.eurpolymj.2022.111454 doi: 10.1016/j.eurpolymj.2022.111454
![]() |
[39] |
Liu Y, Wang L, Mi Y, et al. (2022) Transparent stretchable hydrogel sensors: Materials, design and applications. J Mater Chem C 10: 13351–13371. https://doi.org/10.1039/D2TC01104B doi: 10.1039/D2TC01104B
![]() |
[40] |
Liu G, Chen X, Zhou W, et al. (2016) Preparation of a novel composite nanofiber gel‑encapsulated human placental extract through layer‑by‑layer self‑assembly. Exp Ther Med 11: 1447–1452. https://doi.org/10.3892/etm.2016.3084 doi: 10.3892/etm.2016.3084
![]() |
[41] |
Zhao Z, Fang R, Rong Q, et al. (2017) Bioinspired nanocomposite hydrogels with highly ordered structures. Adv Mater 29: 1703045. https://doi.org/10.1002/adma.201703045 doi: 10.1002/adma.201703045
![]() |
[42] |
Li J, Li S, Huang J, et al. (2022) Spider silk-inspired artificial fibers. Adv Sci 9: 2103965. https://doi.org/10.1002/advs.202103965 doi: 10.1002/advs.202103965
![]() |
[43] |
Zhang X, Wang X, Fan W, et al. (2022) Fabrication, property and application of calcium alginate fiber: A review. Polymers 14: 3227. https://doi.org/10.3390/polym14153227 doi: 10.3390/polym14153227
![]() |
[44] |
Yetisen AK, Jiang N, Fallahi A, et al. (2017) Glucose-sensitive hydrogel optical fibers functionalized with phenylboronic acid. Adv Mater 29: 1606380. https://doi.org/10.1002/adma.201606380 doi: 10.1002/adma.201606380
![]() |
[45] |
Choi M, Humar M, Kim S, et al. (2015) Step-index optical fiber made of biocompatible hydrogels. Adv Mater 27: 4081–4086. https://doi.org/10.1002/adma.201501603 doi: 10.1002/adma.201501603
![]() |
[46] |
Zhang S, Chen Y, Liu H, et al. (2020) Room-temperature-formed PEDOT:PSS hydrogels enable injectable, soft, and healable organic bioelectronics. Adv Mater 32: 1904752. https://doi.org/10.1002/adma.201904752 doi: 10.1002/adma.201904752
![]() |
[47] |
Li Y, Poon CT, Li M, et al. (2015) Chinese-noodle-inspired muscle myofiber fabrication. Adv Funct Mater 25: 5999–6008. https://doi.org/10.1002/adfm.201502018 doi: 10.1002/adfm.201502018
![]() |
[48] |
Dou Y, Wang ZP, He W, et al. (2019) Artificial spider silk from ion-doped and twisted core-sheath hydrogel fibres. Nat Commun 10: 5293. https://doi.org/10.1038/s41467-019-13257-4 doi: 10.1038/s41467-019-13257-4
![]() |
[49] |
Kim SH, Kim SH, Nair S, et al. (2005) Reactive electrospinning of cross-linked poly(2-hydroxyethyl methacrylate) nanofibers and elastic properties of individual hydrogel nanofibers in aqueous solutions. Macromolecules 38: 3719–3723. https://doi.org/10.1021/ma050308g doi: 10.1021/ma050308g
![]() |
[50] |
Jeong W, Kim J, Kim S, et al. (2004) Hydrodynamic microfabrication via "on the fly" photopolymerization of microscale fibers and tubes. Lab Chip 4: 576–580. https://doi.org/10.1039/B411249K doi: 10.1039/B411249K
![]() |
[51] |
Scotti A, Schulte MF, Lopez CG, et al. (2022) How softness matters in soft nanogels and nanogel assemblies. Chem Rev 122: 11675–11700. https://doi.org/10.1021/acs.chemrev.2c00035 doi: 10.1021/acs.chemrev.2c00035
![]() |
[52] |
Ko A, Liao C (2023) Hydrogel wound dressings for diabetic foot ulcer treatment: Status-quo, challenges, and future perspectives. BMEMat 1: e12037. https://doi.org/10.1002/bmm2.12037 doi: 10.1002/bmm2.12037
![]() |
[53] |
Li P, Jin Z, Peng L, et al. (2018) Stretchable all-gel-state fiber-shaped supercapacitors enabled by macromolecularly interconnected 3D graphene/nanostructured conductive polymer hydrogels. Adv Mater 30: 1800124. https://doi.org/10.1002/adma.201800124 doi: 10.1002/adma.201800124
![]() |
[54] |
Perazzo A, Nunes JK, Guido S, et al. (2017) Flow-induced gelation of microfiber suspensions. P Natl Acad Sci USA 114: E8557–E8564. https://doi.org/10.1073/pnas.1710927114 doi: 10.1073/pnas.1710927114
![]() |
[55] |
Kim J, Kang T, Kim H, et al. (2019) Preparation of PVA/PAA nanofibers containing thiol-modified silica particles by electrospinning as an eco-friendly Cu (Ⅱ) adsorbent. J Ind Eng Chem 77: 273–279. https://doi.org/10.1016/j.jiec.2019.04.048 doi: 10.1016/j.jiec.2019.04.048
![]() |
[56] |
Cui Q, Bell DJ, Rauer SB, et al. (2020) Wet-spinning of biocompatible core-shell polyelectrolyte complex fibers for tissue engineering. Adv Mater Interfaces 7: 2000849. https://doi.org/10.1002/admi.202000849 doi: 10.1002/admi.202000849
![]() |
[57] |
Jin Y, Liu C, Chai W, et al. (2017) Self-supporting nanoclay as internal scaffold material for direct printing of soft hydrogel composite structures in air. ACS Appl Mater Interfaces 9: 17456–17465. https://doi.org/10.1021/acsami.7b03613 doi: 10.1021/acsami.7b03613
![]() |
[58] |
Foudazi R, Zowada R, Manas-Zloczower I, et al. (2023) Porous hydrogels: Present challenges and future opportunities. Langmuir 39: 2092–2111. https://doi.org/10.1021/acs.langmuir.2c02253 doi: 10.1021/acs.langmuir.2c02253
![]() |
[59] |
Tamayol A, Najafabadi AH, Aliakbarian B, et al. (2015) Hydrogel templates for rapid manufacturing of bioactive fibers and 3D constructs. Adv Healthc Mater 4: 2146–2153. https://doi.org/10.1002/adhm.201500492 doi: 10.1002/adhm.201500492
![]() |
[60] |
Ding H, Wu Z, Wang H, et al. (2022) An ultrastretchable, high-performance, and crosstalk-free proximity and pressure bimodal sensor based on ionic hydrogel fibers for human-machine interfaces. Mater Horiz 9: 1935–1946. https://doi.org/10.1039/D2MH00281G doi: 10.1039/D2MH00281G
![]() |
[61] |
Yin T, Wu L, Wu T, et al. (2019) Ultrastretchable and conductive core/sheath hydrogel fibers with multifunctionality. J Polym Sci Part B: Polym Phys 57: 272–280. https://doi.org/10.1002/polb.24781 doi: 10.1002/polb.24781
![]() |
[62] |
Wang L, Zhong C, Ke D, et al. (2018) Ultrasoft and highly stretchable hydrogel optical fibers for in vivo optogenetic modulations. Adv Opt Mater 6: 1800427. https://doi.org/10.1002/adom.201800427 doi: 10.1002/adom.201800427
![]() |
[63] |
Cheng J, Jun Y, Qin J, et al. (2017) Electrospinning versus microfluidic spinning of functional fibers for biomedical applications. Biomaterials 114: 121–143. https://doi.org/10.1016/j.biomaterials.2016.10.040 doi: 10.1016/j.biomaterials.2016.10.040
![]() |
[64] |
Ahn SY, Mun CH, Lee SH (2015) Microfluidic spinning of fibrous alginate carrier having highly enhanced drug loading capability and delayed release profile. RSC Adv 5: 15172–15181. https://doi.org/10.1039/C4RA11438H doi: 10.1039/C4RA11438H
![]() |
[65] |
Zhou M, Gong J, Ma J (2019) Continuous fabrication of near-infrared light responsive bilayer hydrogel fibers based on microfluidic spinning. E-Polymers 19: 215–224. https://doi.org/10.1515/epoly-2019-0022 doi: 10.1515/epoly-2019-0022
![]() |
[66] |
Shi X, Ostrovidov S, Zhao Y, et al. (2015) Microfluidic spinning of cell-responsive grooved microfibers. Adv Funct Mater 25: 2250–2259. https://doi.org/10.1002/adfm.201404531 doi: 10.1002/adfm.201404531
![]() |
[67] | Kasper FK, Liao J, Kretlow JD, et al. (1979) Flow Perfusion Culture of Mesenchymal Stem Cells for Bone Tissue Engineering, Cambridge: Harvard Stem Cell Institute. https://doi.org/10.3824/stembook.1.18.1 |
[68] |
Gao Z, Xiao X, Carlo AD, et al. (2023) Advances in wearable strain sensors based on electrospun fibers. Adv Funct Mater 33: 2214265. https://doi.org/10.1002/adfm.202214265 doi: 10.1002/adfm.202214265
![]() |
[69] |
Chen C, Tang J, Gu Y, et al. (2019) Bioinspired hydrogel electrospun fibers for spinal cord regeneration. Adv Funct Mater 29: 1806899. https://doi.org/10.1002/adfm.201806899 doi: 10.1002/adfm.201806899
![]() |
[70] |
Im JS, Bai BC, In SJ, et al. (2010) Improved photodegradation properties and kinetic models of a solar-light-responsive photocatalyst when incorporated into electrospun hydrogel fibers. J Colloid Interf Sci 346: 216–221. https://doi.org/10.1016/j.jcis.2010.02.043 doi: 10.1016/j.jcis.2010.02.043
![]() |
[71] |
Han F, Zhang H, Zhao J, et al. (2012) In situ encapsulation of hydrogel in ultrafine fibers by suspension electrospinning. Polym Eng Sci 52: 2695–2704. https://doi.org/10.1002/pen.23227 doi: 10.1002/pen.23227
![]() |
[72] |
Mirabedini A, Foroughi J, Romeo T, et al. (2015) Development and characterization of novel hybrid hydrogel fibers. Macromol Mater Eng 300: 1217–1225. https://doi.org/10.1002/mame.201500152 doi: 10.1002/mame.201500152
![]() |
[73] |
Song JC, Chen S, Sun LJ, et al. (2020) Mechanically and electronically robust transparent organohydrogel fibers. Adv Mater 32: 1906994. https://doi.org/10.1002/adma.201906994 doi: 10.1002/adma.201906994
![]() |
[74] |
Nechyporchuk O, Yang Nilsson T, Ulmefors H, et al. (2020) Wet spinning of chitosan fibers: Effect of sodium dodecyl sulfate adsorption and enhanced dope temperature. ACS Appl Polym Mater 2: 3867–3875. https://doi.org/10.1021/acsapm.0c00562 doi: 10.1021/acsapm.0c00562
![]() |
[75] |
Konop AJ, Colby RH (1999) Polyelectrolyte charge effects on solution viscosity of poly(acrylic acid). Macromolecules 32: 2803–2805. https://doi.org/10.1021/ma9818174 doi: 10.1021/ma9818174
![]() |
[76] |
Li S, Biswas MC, Ford E (2022) Dual roles of sodium polyacrylate in alginate fiber wet-spinning: Modify the solution rheology and strengthen the fiber. Carbohyd Polym 297: 120001. https://doi.org/10.1016/j.carbpol.2022.120001 doi: 10.1016/j.carbpol.2022.120001
![]() |
[77] |
He Y, Zhang N, Gong Q, et al. (2012) Alginate/graphene oxide fibers with enhanced mechanical strength prepared by wet spinning. Carbohyd Polym 88: 1100–1108. https://doi.org/10.1016/j.carbpol.2012.01.071 doi: 10.1016/j.carbpol.2012.01.071
![]() |
[78] |
Zhang C, Xiao P, Zhang D, et al. (2023) Wet-spinning knittable hygroscopic organogel fibers toward moisture-capture-enabled multifunctional devices. Adv Fiber Mater 5: 588–602. https://doi.org/10.1007/s42765-022-00243-7 doi: 10.1007/s42765-022-00243-7
![]() |
[79] |
Sharma A, Ansari MZ, Cho C (2022) Ultrasensitive flexible wearable pressure/strain sensors: Parameters, materials, mechanisms and applications. Sensor Actuat A-Phys 347: 113934. https://doi.org/10.1016/j.sna.2022.113934 doi: 10.1016/j.sna.2022.113934
![]() |
[80] |
Nasiri S, Khosravani MR (2020) Progress and challenges in fabrication of wearable sensors for health monitoring. Sensor Actuat A-Phys 312: 112105. https://doi.org/10.1016/j.sna.2020.112105 doi: 10.1016/j.sna.2020.112105
![]() |
[81] |
Tian K, Bae J, Bakarich SE, et al. (2017) 3D printing of transparent and conductive heterogeneous hydrogel-elastomer systems. Adv Mater 29: 1604827. https://doi.org/10.1002/adma.201604827 doi: 10.1002/adma.201604827
![]() |
[82] |
Liu C, Wang Z, Wei X, et al. (2021) 3D printed hydrogel/PCL core/shell fiber scaffolds with NIR-triggered drug release for cancer therapy and wound healing. Acta Biomater 131: 314–325. https://doi.org/10.1016/j.actbio.2021.07.011 doi: 10.1016/j.actbio.2021.07.011
![]() |
[83] |
Zheng SY, Shen Y, Zhu F, et al. (2018) Programmed deformations of 3D-printed tough physical hydrogels with high response speed and large output force. Adv Funct Mater 28: 1803366. https://doi.org/10.1002/adfm.201803366 doi: 10.1002/adfm.201803366
![]() |
[84] |
Wu Y, Zhou S, Yi J, et al. (2022) Facile fabrication of flexible alginate/polyaniline/graphene hydrogel fibers for strain sensor. J Eng Fiber Fabr 17. https://doi.org/10.1177/15589250221114641 doi: 10.1177/15589250221114641
![]() |
[85] |
Guo J, Liu X, Jiang N, et al. (2016) Highly stretchable, strain sensing hydrogel optical fibers. Adv Mater 28: 10244–10249. https://doi.org/10.1002/adma.201603160 doi: 10.1002/adma.201603160
![]() |
[86] |
Shi W, Wang Z, Song H, et al. (2022) High-sensitivity and extreme environment-resistant sensors based on PEDOT:PSS@PVA hydrogel fibers for physiological monitoring. ACS Appl Mater Interfaces 14: 35114–35125. https://doi.org/10.1021/acsami.2c09556 doi: 10.1021/acsami.2c09556
![]() |
[87] |
Mian Z, Hermayer KL, Jenkins A (2019) Continuous glucose monitoring: Review of an innovation in diabetes management. Am J Med Sci 358: 332–339. https://doi.org/10.1016/j.amjms.2019.07.003 doi: 10.1016/j.amjms.2019.07.003
![]() |
[88] |
Xie Y, Lu L, Gao F, et al. (2021) Integration of artificial intelligence, blockchain, and wearable technology for chronic disease management: A new paradigm in smart healthcare. Curr Med Sci 41: 1123–1133. https://doi.org/10.1007/s11596-021-2485-0 doi: 10.1007/s11596-021-2485-0
![]() |
[89] |
Heo YJ, Shibata H, Okitsu T, et al. (2011) Long-term in vivo glucose monitoring using fluorescent hydrogel fibers. P Natl Acad Sci USA 108: 13399–13403. https://doi.org/10.1073/pnas.1104954108 doi: 10.1073/pnas.1104954108
![]() |
[90] | Heo YJ, Shibata H, Okitsu T, et al. (2011) Fluorescent hydrogel fibers for long-term in vivo glucose monitoring. 16th International Solid-State Sensors, Actuators and Microsystems Conference, Beijing, China, 2140–214. https://10.1109/TRANSDUCERS.2011.5969342 |
[91] |
Nunoi H, Xie P, Nakamura H, et al. (2022) Treatment with polyethylene glycol-conjugated fungal d-amino acid oxidase reduces lung inflammation in a mouse model of chronic granulomatous disease. Inflammation 45: 1668–1679. https://doi.org/10.1007/s10753-022-01650-z doi: 10.1007/s10753-022-01650-z
![]() |
[92] |
Besir Y, Karaagac E, Kurus M, et al. (2023) The effect of bovine serum albumin-glutaraldehyde and polyethylene glycol polymer on local tissue reaction and inflammation in rabbit carotid artery anastomosis. Vascular 31: 554–563. https://doi.org/10.1177/17085381221075484 doi: 10.1177/17085381221075484
![]() |
[93] |
Zdolsek J, Eaton JW, Tang L (2007) Histamine release and fibrinogen adsorption mediate acute inflammatory responses to biomaterial implants in humans. J Transl Med 5: 31. https://doi.org/10.1186/1479-5876-5-31 doi: 10.1186/1479-5876-5-31
![]() |
[94] |
Bosetti M, Zanardi L, Bracco P, et al. (2003) In vitro evaluation of the inflammatory activity of ultra-high molecular weight polyethylene. Biomaterials 24: 1419–1426. https://doi.org/10.1016/S0142-9612(02)00526-4 doi: 10.1016/S0142-9612(02)00526-4
![]() |
[95] |
Elsherif M, Hassan MU, Yetisen AK, et al. (2019) Hydrogel optical fibers for continuous glucose monitoring. Biosens Bioelectron 137: 25–32. https://doi.org/10.1016/j.bios.2019.05.002 doi: 10.1016/j.bios.2019.05.002
![]() |
[96] |
De Fazio R, De Vittorio M, Visconti P (2021) Innovative IoT solutions and wearable sensing systems for monitoring human biophysical parameters: A review. Electronics 10: 1660. https://doi.org/10.3390/electronics10141660 doi: 10.3390/electronics10141660
![]() |
[97] |
Seshadri DR, Li RT, Voos JE, et al. (2019) Wearable sensors for monitoring the physiological and biochemical profile of the athlete. NPJ Digit Med 2: 72. https://doi.org/10.1038/s41746-019-0150-9 doi: 10.1038/s41746-019-0150-9
![]() |
[98] |
Bandodkar AJ, Jeang WJ, Ghaffari R, et al. (2019) Wearable sensors for biochemical sweat analysis. Annu Rev Anal Chem 12: 1–22. https://doi.org/10.1146/annurev-anchem-061318-114910 doi: 10.1146/annurev-anchem-061318-114910
![]() |
[99] |
Hanlon M, Anderson R (2009) Real-time gait event detection using wearable sensors. Gait Posture 30: 523–527. https://doi.org/10.1016/j.gaitpost.2009.07.128 doi: 10.1016/j.gaitpost.2009.07.128
![]() |
[100] |
Prasanth H, Caban M, Keller U, et al. (2021) Wearable sensor-based real-time gait detection: A systematic review. Sensors 21: 2727. https://doi.org/10.3390/s21082727 doi: 10.3390/s21082727
![]() |
[101] |
Wan F, Ping H, Wang W, et al. (2021) Hydroxyapatite-reinforced alginate fibers with bioinspired dually aligned architectures. Carbohyd Polym 267: 118167. https://doi.org/10.1016/j.carbpol.2021.118167 doi: 10.1016/j.carbpol.2021.118167
![]() |
[102] |
Duan X, Yu J, Zhu Y, et al. (2020) Large-scale spinning approach to engineering knittable hydrogel fiber for soft robots. ACS Nano 14: 14929–14938. https://doi.org/10.1021/acsnano.0c04382 doi: 10.1021/acsnano.0c04382
![]() |
[103] |
Song J, Chen S, Sun L, et al. (2020) Mechanically and electronically robust transparent organohydrogel fibers. Adv Mater 32: 1906994. https://doi.org/10.1002/adma.201906994 doi: 10.1002/adma.201906994
![]() |
[104] |
Hou K, Li Y, Liu Y, et al. (2017) Continuous fabrication of cellulose nanocrystal/poly(ethylene glycol) diacrylate hydrogel fiber from nanocomposite dispersion: Rheology, preparation and characterization. Polymer 123: 55–64. https://doi.org/10.1016/j.polymer.2017.06.034 doi: 10.1016/j.polymer.2017.06.034
![]() |
[105] |
Dzenis Y (2004) Spinning continuous fibers for nanotechnology. Science 304: 1917–1919. https://www.science.org/doi/10.1126/science.1099074 doi: 10.1126/science.1099074
![]() |
[106] |
Zhao L, Xu T, Wang B, et al. (2023) Continuous fabrication of robust ionogel fibers for ultrastable sensors via dynamic reactive spinning. Chem Eng J 455: 140796. https://doi.org/10.1016/j.cej.2022.140796 doi: 10.1016/j.cej.2022.140796
![]() |
[107] |
Pei M, Zhu D, Yang J, et al. (2023) Multi-crosslinked flexible nanocomposite hydrogel fibers with excellent strength and knittability. Eur Polym J 182: 111737. https://doi.org/10.1016/j.eurpolymj.2022.111737 doi: 10.1016/j.eurpolymj.2022.111737
![]() |
[108] |
Hua J, Liu C, Fei B, et al. (2022) Self-healable and super-tough double-network hydrogel fibers from dynamic acylhydrazone bonding and supramolecular interactions. Gels 8: 101. https://doi.org/10.3390/gels8020101 doi: 10.3390/gels8020101
![]() |
[109] |
Wang C, Zhai S, Yuan Z, et al. (2020) Drying graphene hydrogel fibers for capacitive energy storage. Carbon 164: 100–110. https://doi.org/10.1016/j.carbon.2020.03.053 doi: 10.1016/j.carbon.2020.03.053
![]() |
[110] |
Meng F, Ding Y (2011) Sub-micrometer-thick all-solid-state supercapacitors with high power and energy densities. Adv Mater 23: 4098–4102. https://doi.org/10.1002/adma.201101678 doi: 10.1002/adma.201101678
![]() |
[111] |
Yang B, Hao C, Wen F, et al. (2017) Flexible black-phosphorus nanoflake/carbon nanotube composite paper for high-performance all-solid-state supercapacitors. ACS Appl Mater Interfaces 9: 44478–44484. https://doi.org/10.1021/acsami.7b13572 doi: 10.1021/acsami.7b13572
![]() |
[112] |
Zhang X, Chen Y, Yan J, et al. (2020) Janus-faced film with dual function of conductivity and pseudo-capacitance for flexible supercapacitors with ultrahigh energy density. Chem Eng J 388: 124197. https://doi.org/10.1016/j.cej.2020.124197 doi: 10.1016/j.cej.2020.124197
![]() |
[113] |
Han X, Xiao G, Wang Y, et al. (2020) Design and fabrication of conductive polymer hydrogels and their applications in flexible supercapacitors. J Mater Chem A 8: 23059–23095. https://doi.org/10.1039/D0TA07468C doi: 10.1039/D0TA07468C
![]() |
[114] |
Huang H, Han L, Fu X, et al. (2021) A powder self-healable hydrogel electrolyte for flexible hybrid supercapacitors with high energy density and sustainability. Small 17: 2006807. https://doi.org/10.1002/smll.202006807 doi: 10.1002/smll.202006807
![]() |
[115] |
Jia R, Li L, Ai Y, et al. (2018) Self-healable wire-shaped supercapacitors with two twisted NiCo2O4 coated polyvinyl alcohol hydrogel fibers. Sci China Mater 61: 254–262. https://doi.org/10.1007/s40843-017-9177-5 doi: 10.1007/s40843-017-9177-5
![]() |
[116] |
Xu T, Yang D, Zhang S, et al. (2021) Antifreezing and stretchable all-gel-state supercapacitor with enhanced capacitances established by graphene/PEDOT-polyvinyl alcohol hydrogel fibers with dual networks. Carbon 171: 201–210. https://doi.org/10.1016/j.carbon.2020.08.071 doi: 10.1016/j.carbon.2020.08.071
![]() |
[117] |
Cao X, Jiang C, Sun N, et al. (2021) Recent progress in multifunctional hydrogel-based supercapacitors. J Sci-Adv Mater Dev 6: 338–350. https://doi.org/10.1016/j.jsamd.2021.06.002 doi: 10.1016/j.jsamd.2021.06.002
![]() |
[118] |
Liu J, Jia Y, Jiang Q, et al. (2018) Highly conductive hydrogel polymer fibers toward promising wearable thermoelectric energy harvesting. ACS Appl Mater Interfaces 10: 44033–44040. https://doi.org/10.1021/acsami.8b15332 doi: 10.1021/acsami.8b15332
![]() |
[119] |
Zhou M, Al-Furjan MSH, Zou J, et al. (2018) A review on heat and mechanical energy harvesting from human-principles, prototypes and perspectives. Renew Sust Energ Rev 82: 3582–3609. https://doi.org/10.1016/j.rser.2017.10.102 doi: 10.1016/j.rser.2017.10.102
![]() |
[120] |
Huang L, Lin S, Xu Z, et al. (2020) Fiber-based energy conversion devices for human-body energy harvesting. Adv Mater 32: 1902034. https://doi.org/10.1002/adma.201902034 doi: 10.1002/adma.201902034
![]() |
[121] |
Peng L, Liu Y, Huang J, et al. (2018) Microfluidic fabrication of highly stretchable and fast electro-responsive graphene oxide/polyacrylamide/alginate hydrogel fibers. Eur Polym J 103: 335–341. https://doi.org/10.1016/j.eurpolymj.2018.04.019 doi: 10.1016/j.eurpolymj.2018.04.019
![]() |
[122] |
Wang Y, Ding Y, Guo X, et al. (2019) Conductive polymers for stretchable supercapacitors. Nano Res 12: 1978–1987. https://doi.org/10.1007/s12274-019-2296-9 doi: 10.1007/s12274-019-2296-9
![]() |
[123] |
Zhao X, Chen F, Li Y, et al. (2018) Bioinspired ultra-stretchable and anti-freezing conductive hydrogel fibers with ordered and reversible polymer chain alignment. Nat Commun 9: 3579. https://doi.org/10.1038/s41467-018-05904-z doi: 10.1038/s41467-018-05904-z
![]() |
[124] |
Bai J, Liu D, Tian X, et al. (2022) Tissue-like organic electrochemical transistors. J Mater Chem C 10: 13303–13311. https://doi.org/10.1039/D2TC01530G doi: 10.1039/D2TC01530G
![]() |
[125] |
Kayser LV, Lipomi DJ (2019) Stretchable conductive polymers and composites based on PEDOT and PEDOT:PSS. Adv Mater 31: 1806133. https://doi.org/10.1002/adma.201806133 doi: 10.1002/adma.201806133
![]() |
[126] |
Tseghai GB, Mengistie DA, Malengier B, et al. (2020) PEDOT:PSS-based conductive textiles and their applications. Sensors 20: 1881. https://doi.org/10.3390/s20071881 doi: 10.3390/s20071881
![]() |
[127] |
Zhao P, Zhang R, Tong Y, et al. (2020) Strain-discriminable pressure/proximity sensing of transparent stretchable electronic skin based on PEDOT:PSS/SWCNT electrodes. ACS Appl Mater Interfaces 12: 55083–55093. https://doi.org/10.1021/acsami.0c16546 doi: 10.1021/acsami.0c16546
![]() |
[128] |
Li P, Wang Y, Gupta U, et al. (2019) Transparent soft robots for effective camouflage. Adv Funct Mater 29: 1901908. https://doi.org/10.1002/adfm.201901908 doi: 10.1002/adfm.201901908
![]() |
[129] |
Li Y, Wang J, Wang Y, et al. (2021) Advanced electrospun hydrogel fibers for wound healing. Compos Part B-Eng 223: 109101. https://doi.org/10.1016/j.compositesb.2021.109101 doi: 10.1016/j.compositesb.2021.109101
![]() |
[130] |
Neibert K, Gopishetty V, Grigoryev A, et al. (2012) Wound-healing with mechanically robust and biodegradable hydrogel fibers loaded with silver nanoparticles. Adv Healthc Mater 1: 621–630. https://doi.org/10.1002/adhm.201200075 doi: 10.1002/adhm.201200075
![]() |
[131] |
Peng J, Cheng Q (2017) High-performance nanocomposites inspired by nature. Adv Mater 29: 1702959. https://doi.org/10.1002/adma.201702959 doi: 10.1002/adma.201702959
![]() |
[132] |
Xia LW, Xie R, Ju XJ, et al. (2013) Nano-structured smart hydrogels with rapid response and high elasticity. Nat Commun 4: 2226. https://doi.org/10.1038/ncomms3226 doi: 10.1038/ncomms3226
![]() |
[133] |
Lan Z, Kar R, Chwatko M, et al. (2023) High porosity PEG-based hydrogel foams with self-tuning moisture balance as chronic wound dressings. J Biomed Mater Res A 111: 465–477. https://doi.org/10.1002/jbm.a.37498 doi: 10.1002/jbm.a.37498
![]() |
[134] |
Farahani M, Shafiee A (2021) Wound healing: From passive to smart dressings. Adv Healthc Mater 10: 2100477. https://doi.org/10.1002/adhm.202100477 doi: 10.1002/adhm.202100477
![]() |
[135] |
Zhang Q, Qian C, Xiao W, et al. (2019) Development of a visible light, cross-linked GelMA hydrogel containing decellularized human amniotic particles as a soft tissue replacement for oral mucosa repair. RSC Adv 9: 18344–18352. https://doi.org/10.1039/C9RA03009C doi: 10.1039/C9RA03009C
![]() |
[136] |
Raho R, Paladini F, Lombardi FA, et al. (2015) In-situ photo-assisted deposition of silver particles on hydrogel fibers for antibacterial applications. Mater Sci Eng C 55: 42–49. https://doi.org/10.1016/j.msec.2015.05.050 doi: 10.1016/j.msec.2015.05.050
![]() |
[137] |
Ji Y, Ghosh K, Li B, et al. (2006) Dual-syringe reactive electrospinning of cross-linked hyaluronic acid hydrogel nanofibers for tissue engineering applications. Macromol Biosci 6: 811–817. https://doi.org/10.1002/mabi.200600132 doi: 10.1002/mabi.200600132
![]() |
[138] |
Sun X, Lang Q, Zhang H, et al. (2017) Electrospun photocrosslinkable hydrogel fibrous scaffolds for rapid in vivo vascularized skin flap regeneration. Adv Funct Mater 27: 1604617. https://doi.org/10.1002/adfm.201604617 doi: 10.1002/adfm.201604617
![]() |
[139] |
Liu W, Bi W, Sun Y, et al. (2020) Biomimetic organic-inorganic hybrid hydrogel electrospinning periosteum for accelerating bone regeneration. Mater Sci Eng C 110: 110670. https://doi.org/10.1016/j.msec.2020.110670 doi: 10.1016/j.msec.2020.110670
![]() |
[140] |
Wei X, Liu C, Wang Z, et al. (2020) 3D printed core-shell hydrogel fiber scaffolds with NIR-triggered drug release for localized therapy of breast cancer. Int J Pharm 580: 119219. https://doi.org/10.1016/j.ijpharm.2020.119219 doi: 10.1016/j.ijpharm.2020.119219
![]() |
[141] |
Tsourdi E, Barthel A, Rietzsch H, et al. (2013) Current aspects in the pathophysiology and treatment of chronic wounds in diabetes mellitus. Biomed Res Int 2013: 385641. https://doi.org/10.1155/2013/385641 doi: 10.1155/2013/385641
![]() |
[142] |
Chen H, Jia P, Kang H, et al. (2016) Upregulating hif-1α by hydrogel nanofibrous scaffolds for rapidly recruiting angiogenesis relative cells in diabetic wound. Adv Healthc Mater 5: 907–918. https://doi.org/10.1002/adhm.201501018 doi: 10.1002/adhm.201501018
![]() |
[143] |
Zhou P, Zhou L, Zhu C, et al. (2016) Nanogel-electrospinning for controlling the release of water-soluble drugs. J Mater Chem B 4: 2171–2178. https://doi.org/10.1039/C6TB00023A doi: 10.1039/C6TB00023A
![]() |
[144] |
Zhao X, Sun X, Yildirimer L, et al. (2017) Cell infiltrative hydrogel fibrous scaffolds for accelerated wound healing. Acta Biomater 49: 66–77. https://doi.org/10.1016/j.actbio.2016.11.017 doi: 10.1016/j.actbio.2016.11.017
![]() |
[145] |
Wang H, Xu Z, Zhao M, et al. (2021) Advances of hydrogel dressings in diabetic wounds. Biomater Sci 9: 1530–1546. https://doi.org/10.1039/D0BM01747G doi: 10.1039/D0BM01747G
![]() |
[146] |
Lei H, Zhu C, Fan D (2020) Optimization of human-like collagen composite polysaccharide hydrogel dressing preparation using response surface for burn repair. Carbohyd Polym 239: 116249. https://doi.org/10.1016/j.carbpol.2020.116249 doi: 10.1016/j.carbpol.2020.116249
![]() |
[147] |
Kesharwani P, Bisht A, Alexander A, et al. (2021) Biomedical applications of hydrogels in drug delivery system: An update. J Drug Deliv Sci Tec 66: 102914. https://doi.org/10.1016/j.jddst.2021.102914 doi: 10.1016/j.jddst.2021.102914
![]() |
[148] |
Kamoun EA, Kenawy ERS, Chen X (2017) A review on polymeric hydrogel membranes for wound dressing applications: PVA-based hydrogel dressings. J Adv Res 8: 217–233. https://doi.org/10.1016/j.jare.2017.01.005 doi: 10.1016/j.jare.2017.01.005
![]() |
[149] |
Ahmad F, Mushtaq B, Butt FA, et al. (2021) Synthesis and characterization of nonwoven cotton-reinforced cellulose hydrogel for wound dressings. Polymers 13: 4098. https://doi.org/10.3390/polym13234098 doi: 10.3390/polym13234098
![]() |
[150] |
Wang W, Zeng Z, Xiang L, et al. (2021) Injectable self-healing hydrogel via biological environment-adaptive supramolecular assembly for gastric perforation healing. ACS Nano 15: 9913–9923. https://doi.org/10.1021/acsnano.1c01199 doi: 10.1021/acsnano.1c01199
![]() |
[151] |
Yao Y, Zhang A, Yuan C, et al. (2021) Recent trends on burn wound care: Hydrogel dressings and scaffolds. Biomater Sci 9: 4523–4540. https://doi.org/10.1039/D1BM00411E doi: 10.1039/D1BM00411E
![]() |
[152] |
Jiang S, Deng J, Jin Y, et al. (2023) Breathable, antifreezing, mechanically skin-like hydrogel textile wound dressings with dual antibacterial mechanisms. Bioact Mater 21: 313–323. https://doi.org/10.1016/j.bioactmat.2022.08.014 doi: 10.1016/j.bioactmat.2022.08.014
![]() |
[153] |
Liu J, Zhang C, Miao D, et al. (2018) Preparation and characterization of carboxymethylcellulose hydrogel fibers. J Eng Fiber Fabr 13. https://doi.org/10.1177/155892501801300302 doi: 10.1177/155892501801300302
![]() |
[154] |
Dong Y, Zheng Y, Zhang K, et al. (2020) Electrospun nanofibrous materials for wound healing. Adv Fiber Mater 2: 212–227. https://doi.org/10.1007/s42765-020-00034-y doi: 10.1007/s42765-020-00034-y
![]() |
1. | Lathika Vaniyan, Pallab Kumar Borah, Galina E. Pavlovskaya, Nick Terrill, Joshua E. S. J. Reid, Michael Boehm, Philippe Prochasson, Reed A. Nicholson, Stefan Baier, Gleb E. Yakubov, Wet spinning of sodium carboxymethyl cellulose–sodium caseinate hydrogel fibres: relationship between rheology and spinnability, 2025, 1744-683X, 10.1039/D4SM00705K |