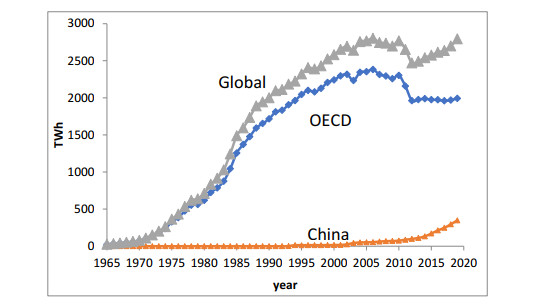
Nuclear energy currently accounts for a declining share of global electricity, but it is possible that rising concerns about global climate change and China's ambitious nuclear program could reverse this trend. This review attempts to assess the global future of nuclear power, showing how the optimistic forecasts in the early days of nuclear power have been replaced by far more modest forecasts. The review first discusses the controversies surrounding nuclear power. It then briefly examines the prospects for three proposed reactors of the future: Small Modular Reactors; Generation IV breeder reactors; fusion reactors. It finally discusses the social and political context for nuclear power, both today and in the future.
Citation: Patrick Moriarty. Global nuclear energy: an uncertain future[J]. AIMS Energy, 2021, 9(5): 1027-1042. doi: 10.3934/energy.2021047
[1] | Emmanuel Arthur . Energy development: A global perspective and advances in Ghana. AIMS Energy, 2022, 10(2): 306-339. doi: 10.3934/energy.2022017 |
[2] | Patrick Moriarty, Damon Honnery . The limits of renewable energy. AIMS Energy, 2021, 9(4): 812-829. doi: 10.3934/energy.2021037 |
[3] | Patrick Moriarty, Damon Honnery . Energy policy and economics under climate change. AIMS Energy, 2018, 6(2): 272-290. doi: 10.3934/energy.2018.2.272 |
[4] | Ahmad Indra Siswantara, Illa Rizianiza, Tanwir Ahmad Farhan, M. Hilman Gumelar Syafei, Dyas Prawara Mahdi, Candra Damis Widiawaty, Adi Syuriadi . Analyzing temperature distribution in pyrolysis systems using an atomic model. AIMS Energy, 2023, 11(6): 1012-1030. doi: 10.3934/energy.2023048 |
[5] | Shahrouz Abolhosseini, Almas Heshmati, Masoomeh Rashidghalam . Energy security and competition over energy resources in Iran and Caucasus region. AIMS Energy, 2017, 5(2): 224-238. doi: 10.3934/energy.2017.2.224 |
[6] | Chukwuebuka Okafor, Christian Madu, Charles Ajaero, Juliet Ibekwe, Happy Bebenimibo, Chinelo Nzekwe . Moving beyond fossil fuel in an oil-exporting and emerging economy: Paradigm shift. AIMS Energy, 2021, 9(2): 379-413. doi: 10.3934/energy.2021020 |
[7] | David Schwartzman, Peter Schwartzman . Scenarios for combating global warming: China's critical role as a leader in the energy transition. AIMS Energy, 2024, 12(4): 809-821. doi: 10.3934/energy.2024038 |
[8] | Nour Khlaifat, Ali Altaee, John Zhou, Yuhan Huang . A review of the key sensitive parameters on the aerodynamic performance of a horizontal wind turbine using Computational Fluid Dynamics modelling. AIMS Energy, 2020, 8(3): 493-524. doi: 10.3934/energy.2020.3.493 |
[9] | Khuthadzo Kgopana, Olawale Popoola . Improved utilization of hybrid energy for low-income houses based on energy consumption pattern. AIMS Energy, 2023, 11(1): 79-109. doi: 10.3934/energy.2023005 |
[10] | Akram Jahanshahi, Dina Jahanianfard, Amid Mostafaie, Mohammadreza Kamali . An Auto Regressive Integrated Moving Average (ARIMA) Model for prediction of energy consumption by household sector in Euro area. AIMS Energy, 2019, 7(2): 151-164. doi: 10.3934/energy.2019.2.151 |
Nuclear energy currently accounts for a declining share of global electricity, but it is possible that rising concerns about global climate change and China's ambitious nuclear program could reverse this trend. This review attempts to assess the global future of nuclear power, showing how the optimistic forecasts in the early days of nuclear power have been replaced by far more modest forecasts. The review first discusses the controversies surrounding nuclear power. It then briefly examines the prospects for three proposed reactors of the future: Small Modular Reactors; Generation IV breeder reactors; fusion reactors. It finally discusses the social and political context for nuclear power, both today and in the future.
Abbreviations: AEC: Atomic Energy Agency (US); EJ: exajoule = 1018 joule; FF: fossil fuels; EROI: energy return on energy invested; GHG: greenhouse gas; GW: gigawatt (109 watt); IAEA: International Atomic Energy Agency; IPCC: Intergovernmental Panel on Climate Change; ITER: International Thermonuclear Experimental Reactor; LWR: Light Water Reactor; MW: megawatt (106 watt); OECD: Organization for Economic Cooperation and Development; ppm: parts per million; RE: renewable energy; SMR: Small Modular Reactor; TWh: terawatt-hour (1012 watt-hour)
The first nuclear power plant was connected to the electricity grid in 1956 in the UK. The 1960s and 1970s were times of unbridled optimism for the future of nuclear power. In a 1976 publication, the International Atomic Energy Agency (IAEA) [1] predicted a global nuclear installed capacity of 2500 gigawatt (GW) for year 2000. Nor were the IAEA alone in forecasts of this type. For the US, Lane [2] in 1959 forecast 600 GW nuclear capacity, with over 30% coming from breeder reactors. Again, for the US, the Atomic Energy Agency (AEC) and Energy Research and Development Agency (ERDA) issued predictions for installed nuclear capacity for the year 2000. Predicted values for year 2000 rose in the late-1960s before peaking in the early years of the 1970s at between 1200 and 1500 GW, before falling steeply after around 1973 [3]. Joskow and Baughman [4] also forecast high penetration of nuclear power in the US by 1995, with most scenario values in the 400-600 GW range.
The actual installed capacity in 2000 was only 348 GW globally, and around 95 GW for the US [5]. Global nuclear power output did rise quite rapidly until the late 1980s, but then the annual growth rate fell, as shown in Figure 1. As a share of global electricity, nuclear power peaked at 17.5% as early as 1996, but by 2017 had fallen to only 10.3% [6]. In absolute output, nuclear energy in 2019 and 2020 had not yet surpassed the 2006 value of 2804 terawatt-hours (TWh).
As shown in Figure 1, the OECD countries still produce most of the world's nuclear power, but their share is falling. The share of nuclear power in electricity production varies widely between countries. For most countries it is zero. In France, however, it is over 70% and over 50% in the Slovak Republic and Hungary. In India and China with ambitious nuclear plans, nuclear power's share is still only a few percent [7].
Why were the forecasts for nuclear energy futures so optimistic in the 1970s? One possible reason is simple economics: nuclear proponents believed that the nuclear electricity option would be far cheaper than electricity from fossil fuels. In the often-quoted words of the chairman of the US (AEC) in 1954, nuclear electricity would be 'too cheap to meter' [8]. An important reason for this low predicted cost was the small fuel load for reactors (and small size of reactors themselves) compared with coal power stations of the same output. (Another possible reason was the reaction of many nuclear physicists to the nuclear bombs dropped on Japan in 1945. Nuclear power offered the chance of turning 'swords into plough shares'. Unfortunately, the record shows that some countries found ways of turning the ploughshares back into nuclear weapons).
As Figure 1 shows, in the OECD countries, which still account for most nuclear energy, output is in decline, the result of opposition to nuclear power and (probably related) escalating costs of construction. China, and to a lesser extent other rapidly industrializing countries, are seen by the industry as providing the main hope for a nuclear power revival. Particularly in OECD countries, nuclear reactors have taken many years to plan and build, partly because of their complexity, and partly because of a lack of standardization. Further, as a result of the downturn in new reactor start-ups, the world's reactor fleet is ageing [9], and many are planned to be shut down in the next decade or so [10]. The result of long construction lead times is that forecasting the maximum possible nuclear output is relatively simple over the next decade or so. After that period, the nuclear future is increasingly uncertain.
Figure 2 plots the percentage of all articles on energy/fuel in the Elsevier Scopus database that have the term 'nuclear energy' in the title, abstract or keywords. This percentage reached a peak of over 60% in 1970, before falling rapidly in the early 1970s, and today is under 2%. Overall, articles with either of the general terms, 'energy' or 'fuel' in the title, abstract or keywords have risen from under 500 in 1970 to over 130, 000 in 2020. The nuclear share of articles in 1970 has been steadily replaced by articles on renewable energy sources, particularly solar, wind and bioenergy. This result strongly suggests that research interest in nuclear energy is also losing momentum.
Although Figure 2 shows a relative decline in nuclear power's share of energy papers of all types, there were still over six thousand reviewed articles on nuclear power published in the three years 2018-2020. Clearly, only some could be included for review. In general, more recent papers were favoured, but because the history of nuclear power is also important, selected articles from several decades ago were also included. Further, given the polarisation of views on nuclear power, papers from both 'sides' of the debate were discussed, as shown in Table 1, discussed below. Finally, narrowly technical papers were excluded; technical detail has only been supplied to the extent needed for understanding the issues involved. What is also novel in this review is its global, future emphasis, and the attempt to situate nuclear energy not only among competing energy sources, but also to discuss this energy future in the changed world thrown up by climate change and the current pandemic.
Nuclear Energy controversy | 'Optimistic' papers | 'Pessimistic' papers |
Nuclear power's future | [1,2,3,4] | [13,21,22,23,24,25] |
Nuclear energy essential for climate change mitigation | [14,15,16,17,26] | [25,27,28] |
Energy return on energy invested (EROI) for nuclear energy | [29,30] | [5,31] |
Future costs for nuclear energy | [32] | [10,33] |
Size and cost of uranium reserves | [34,35] | [36] |
Environmental benefits of nuclear power | [16] | [37] |
Dangers of nuclear weapons proliferation | [38] | [5,23] |
Risk from serious nuclear accidents | [34] | [39,40] |
Health effects of low-level radiation | [41] | [42] |
Successful introduction of fusion power | [15,43,44] | [45,46,47] |
The rest of this review is organised as follows. The next section looks at the host of controversies that have surrounded nuclear power since its inception. Although all energy generation can produce controversies and opposition, some are unique to nuclear energy. Section 3 'Future Nuclear Power: Technology' examines three technologies for future nuclear power: small modular reactors, breeder reactors, and fusion energy. None are new proposals, and only fusion energy has no history of commercial energy generation. The section 'Future Nuclear Power: Social, Economic and Political' discusses the non-technical aspects of nuclear energy, as these will have a vital role in the success or failure of the nuclear enterprise. The section 'Discussion' offers an overview of the main findings and a very tentative view of nuclear energy's future prospects. A very brief 'Conclusions' section gives a summary of the main points.
Over the past four decades or so, nuclear energy has generated extraordinary controversy, both at the popular level and in the published literature. As Machin [11] stated: '[…] nuclear power is simultaneously regarded both as the best means of addressing climate change and as the worst'. All energy sources, including fossil fuels (FFs) and renewable energy (RE) sources, have at least some adverse effects, and all receive subsidies to some extent [12]. The adverse environmental effects for large-scale hydroelectric projects, for example, are well-known. While some nuclear power countries in the OECD are committed to closing all nuclear plants in view of nuclear power's perceived adverse effects, other nations, particularly China (see Figure 1), are pushing ahead with ambitious nuclear programs.
Table 1 gives a small selection of mainly recently published papers from the vast literature on both sides of the controversy for a number of different aspects of nuclear power. Most of the authors listed in one or other entries in the 'Optimistic' column would also be optimistic on the other entries; the same is true for 'Pessimistic' column authors. Understandably, articles from those working in the nuclear industry or in nuclear engineering university departments tend to be optimistic about nuclear energy. More generalist energy researchers are far more evenly divided in their support 'for' or 'against' nuclear power. Although there are more conflicting views on nuclear power than on other energy sources, controversies and disagreements among experts do appear to be a feature of energy research in general [13].
One overarching problem facing nuclear power is that for success, nuclear power must score positively on most of the controversies listed in Table 1, whereas it might only take one negative entry to doom this energy source. If the risk of serious nuclear accidents is too great, if uranium reserves are not adequate, or if costs are much greater than alternative energy sources (such as renewable energy), then nuclear power's future is in doubt.
Possibly the most important argument in favour of expanding nuclear power is that nuclear power is essential for climate change mitigation [14,15,16,17]. At present, despite the rhetoric, climate change is not taken seriously enough to affect emissions; fossil fuel CO2 emissions and resulting atmospheric CO2 levels are still growing strongly [6,17]. But such neglect cannot continue for much longer, so that many researchers think that all alternatives to fossil fuels must be considered. Further, massive and rapid expansion of the other non-carbon energy source, renewable energy, has its own problems [18,19]. Nuclear power may not be a renewable energy source, but is certainly a low-carbon energy source: this much is acknowledged by nearly all energy researchers. A number of researchers [e.g., 20] have argued that nuclear and renewable energy can complement each other to produce all energy needs, not just electricity.
One of the difficulties facing nuclear power is the lack of standardisation for reactor designs. Unlike, for example, hydroelectric power stations, which must be adapted to the characteristics of the site, there is little reason why reactor designs should vary so widely. Most nuclear plants today—for example, the common light water reactors (LWRs)—are thermal reactors. Such reactors use so-called slow or thermal neutrons. 'A thermal moderator is used to slow the neutrons emitted by fission to make them more likely to be captured by the fuel' [48]. The majority of reactors operating today use uranium enriched to 2-4% of the uranium-235 isotope (U-235), which, unlike the far more naturally more abundant U-238, is fissile. Today the gas centrifuge method is preferred for enrichment, because of its much lower energy needs compared with the earlier gas diffusion method.
Most reactors today also use water as coolant and moderator. Others, like the UK Magnox reactors, use unenriched uranium, which only contains 0.7% of the U-235 isotope, as well as graphite as moderator and CO2 as coolant. The Canadian CANDU reactors also use unenriched uranium, but then use heavy water (made from the deuterium hydrogen isotope) for moderator and coolant. CANDU reactors can also be refuelled online, but most thermal reactors, reflecting their naval reactor origins, must be shut down for refuelling. The common use of water for coolant, and compact, high energy density design, also reflects their naval reactor origin.
The reactors being built today are so-called 'Generation III or III+' reactors, which are essentially improved and potentially safer versions of existing LWRs [48], which still have many safety problems [49]. Generation IV reactors are under development, but not expected to be deployed until after 2030, with several of them breeder reactors, discussed below. In this section, three reactors of the future are discussed, but both Small Modular Reactors (SMRs) and breeder reactors have been around for many decades, and have even seen commercial operation. Fusion energy has also been discussed and researched for many decades, but no commercial, or even demonstration plant, is yet in operation.
One response to the long lead times and high costs of conventional reactors is to develop Small Modular Reactors (SMRs), defined as reactors with a rated output of 300 GW or less. They are not really a new idea—interest in them goes back to the 1950s, with a 'second wave of enthusiasm' [50] in the 1980s, but no designs were carried through to the commercial stage. According to Mignacca et al. [51], some 50 different SMR designs are now under development in several countries. The proposed new reactors would be largely factory-made and delivered to site, unlike present large reactors, which can have an output of 1000 megawatt (MW) or more. These SMRs, typically sized 10-100 MW, could be used in remote locations, or in countries or regions with electricity demand too small to support a large conventional reactor [52]. Unlike present large reactors, which are individually designed, the small reactors could potentially be mass-produced. Construction times at the site would be greatly reduced, as the module components would be shipped to the reactor site.
Extravagant claims have been made for these proposed reactors in terms of their safety and utility. SMRs, their advocates argue, could be used for saltwater desalination, and for space exploration [50]. To justify the shift away from conventional large reactors, their proponents acknowledge that present conventional reactors carry significant accident risks and waste disposal problems, but claim that SMRs can overcome these challenges. Cost of electricity might be expected to be higher than that for large reactors, because of loss of economies of scale. For industrial plant in general, construction costs per unit of output rise much more slowly with larger plant size [33]. However, modelled results [53] indicate that costs per MW would be similar to conventional reactors, even considering the cost overruns these have often experienced.
A proposed variant of SMRs is the micro-reactor or 'nuclear battery', with an output of 1-20 MW. Once the battery is exhausted it would be sent back to the manufacturing plant (by truck or shipping container) for refuelling and maintenance [54]. Testoni et al. [55] reported that for such microreactors: 'The main advantages are the small size, the simple plant layout and the fast, on-site installation. The main challenges are the limited fuel availability, the security and proliferation risk and the licensing process'. They also argued that such reactors, while not cost competitive with large conventional reactors, can compete with alternatives such as diesel generators for small-scale applications.
It was realised very early on that thermal reactors using enriched uranium without recycling could not provide for unlimited energy, because of eventual depletion of uranium reserves. For that breeder reactors—or fusion energy, discussed below—were needed [56]. In breeder (or fast) reactors some of the fertile (and abundant) U-238 isotope is converted to plutonium-239 (Pu-239), which like U-235, is also fissile. The difficulty is that to extract this fissile material, the spent fuel must be reprocessed, a complex and expensive process. Since Pu-239 is a weapons grade material, widespread reprocessing under cover of a civilian breeder reactor program would raise the risk of nuclear weapons proliferation in an already deeply-divided world. Nor are countries aspiring to nuclear power likely to accede to proposals to confine spent nuclear fuel reprocessing to a handful of countries such as France, Japan and the US.
One of the proposed Generation IV reactor designs is the sodium-cooled fast breeder reactor. As with SMRs, this type of nuclear reactor has been around for decades, and several have operated commercially. As Locatelli et al. [57] state in their review of Generation IV designs: 'The main advantage of this technology is that the fast spectrum is able to convert fertile material into fissile, increasing the efficiency of usage of the nuclear fuel by about 50 times'. However, water can no longer be used as a coolant. Instead, molten metal is often used as coolant, particularly sodium. Sodium is an excellent coolant, having both a low melting point and a high specific heat. Molten salts have also been employed as coolants. Like nuclear reactor design in general, there is no consensus as to what would be the best breeder reactor design. This lack of consensus stands in marked contrast to wind turbines for example, where the two or three blade horizontal axis design is nearly universal. In recognition of this problem, Generation IV has concentrated on only a handful of the more promising reactor designs. Nevertheless, there has been a series of closures of existing breeder reactors over the last decade or two [58].
Fusion reactors, like SMRs, are advocated by their supporters as overcoming the acknowledged problems facing conventional reactors. Since no fusion reactors are in operation, they can claim a spotless operating and safety record! Half a century ago, Post [44] predicted that fusion energy could see commercial application as early as the mid-1980s. Today, even fusion supporters do not see technical feasibility established before 2035, or its widespread commercial use until at best until much later in the century. If the world is to avoid the worst effects of climate change, decisive action cannot wait until late in the 21st century [17,59].
The tokamak magnetic confinement system is the leading design for commercial fusion power, as used in the International Thermonuclear Experimental Reactor (ITER) plant presently under construction in France. Hirsch [60] has argued that such designs fail on the three criteria for practical fusion power: 'attractive economics, regulatory simplicity, and public acceptance'. For example, the ITER plant has exceeded initial cost estimates by an order of magnitude [61]. Jassby [47], a retired fusion researcher, even entitled his article 'ITER is a showcase... for the drawbacks of fusion energy'. He summarizes the difficulties facing nuclear energy as follows: 'The underlying problem is that all nuclear energy facilities—whether fission or fusion—are extraordinarily complex and exorbitantly expensive'.
Can fusion energy, the universe's fundamental energy source, be considered a truly renewable energy source? Although, unlike U-235, one of its input fuels—the hydrogen isotope deuterium (D) is plentiful. The other input fuel is the hydrogen isotope tritium (T), which has a short half-life, can be bred from lithium. In contrast, the specialist materials needed to contain the hot plasma (at 100 million K) may be in short supply. The confinement materials will become heavily radioactive under the barrage of neutrons produced by the D-T reactions [60]. Hassanein and Sizyuk [61] have pointed out design flaws in ITER-type devices under instabilities and loss of confinement of the hot plasma. This possible loss of confinement will necessitate a strong containment around the reactor core to protect plant workers and the public from radiation releases [60].
This review is about the future of nuclear power. It may be that, given the impacts brought about by climate change (along with the Covid-19 pandemic), the past will be a poor guide to our energy future. The latest Intergovernmental Panel on Climate Change (IPCC) report, released in August 2021 [17] is unequivocal about the urgent need to tackle climate change if we are to avoid disaster. Proposed energy solutions available only in two decades or more, are not useful in avoiding further shifts to extreme weather events even more catastrophic than the wildfires, floods and heatwaves experienced in 2021. Certainly, as discussed in the Introduction, historical nuclear forecasts were wildly optimistic. In this section, the heavily interconnected social, economic and political aspects of nuclear energy are discussed. In this section, four key factors affecting the global future of nuclear power are discussed in the following three sub-sections.
In a general paper on energy futures, Sovacool et al. [62] call for a social science perspective in future energy research. They argue for the need to focus on pressing problems, and for research projects to include authors from a variety of disciplines and geographical backgrounds. They also urge energy researchers to reflect on their own biases and limitations.
Nuclear power must compete economically with alternative methods of power generation. One problem that arises is that all energy sources, not only FFs, but also RE and nuclear power, receive significant subsidies in several forms [63]. Energy policy today is being pulled in two opposite directions. Utility companies and governments favour using the cheapest power generation sources. Since they can presently largely ignore the climate change costs of fossil fuels, such fuels are still favoured, especially outside the OECD countries. However, after decades of inaction on climate change, many countries, especially in the OECD, plan to reduce their CO2 emissions. If substantial carbon taxes are levied to achieve this, costs of fossil fuel power will rise and all low-carbon sources, including nuclear, should benefit.
The cost of nuclear energy may seem a purely technical issue, but in fact this topic cannot be separated from the politics of nuclear power. Political pressures to improve reactor safety (and the safety of the entire nuclear energy cycle, from mining through to radioactive waste disposal), and to minimise the risk of weapons proliferation, have driven up the costs of nuclear power, in OECD countries, at least. The result is that electricity utilities in these countries, even those without a formal commitment to phase out nuclear power, increasingly view new reactors as a financially risky investment. As the IEA [7] have reported for new reactors: 'The main obstacles relate to the sheer scale of investment and long lead times; the risk of construction problems, delays and cost overruns; and the possibility of future changes in policy or the electricity system itself. There have been long delays in completing advanced reactors that are still being built in Finland, France and the United States'. Wealer et al. [64], in a recent paper, report on modelling costs for Generation III reactors, and simply concluded that 'the economics of nuclear power plants are not favourable to future investments, even though additional costs (decommissioning, long-term storage) and the social costs of accidents are not even considered'.
According to M.V. Ramana, cited in Anderson [65], nuclear power is 'the one tech where the costs have gone up, not down, with experience'. Ramana add that: 'The way to think about it is that the more experience we have with nuclear power, the more we learn about potential vulnerabilities that can lead to catastrophic accidents'. Cost overruns are the norm. In the US, spurred on by the Energy Policy Act of 2005, construction started on two new nuclear power plants in Georgia and South Carolina, using the Advanced Passive 1000 design. The South Carolina plant was abandoned in 2017 after $9 billion expenditure. The initial cost estimate for the Georgia plant was $14 billion with a 2016-2017 start-up date, but a recent cost estimate is $29 billion, and is still under construction in 2021 [33].
One approach advocated by the IEA and others to improve the prospects for nuclear energy is to extend the life of existing reactors. As already noted, many reactors are approaching the end of their service lives. Refurbishing these reactors to extend their lives by two decades or more is far cheaper than replacing them with new reactors. It also postpones the decommissioning of old reactors, which is an expensive process. Lifetime extensions by replacing key reactor components still entail investment, but are better able to compete with RE or natural gas generated electricity on cost [7]. However, already in the US, over 90% of nuclear plants have had their operating licenses extended from the usual 40 years, to 60 years [9].
Nuclear power shares a vital property with fossil fuels: its continued use could mortgage the future, placing unfair burdens on future generations. CO2 is a long-lived greenhouse gas (GHG) in the atmosphere, which means that high atmospheric concentrations will persist, even if we stopped emitting CO2 now. Atmospheric CO2 concentrations have now reached 410 ppm—the highest level for millennia—and the world is already experiencing an increase in the frequency and intensity of extreme weather events such as floods and heat waves [16,17,59]. Since further rises in atmospheric CO2 ppm seem inevitable, our legacy to future generations will be an increasingly hostile environment.
However, nuclear wastes are similarly long lived. Plutonium has a half-life of 22, 400 years, which means that a roughly 1000-fold decay needed to render wastes harmless to humans will take 10 half-lives or 224, 000 years [5]. For comparison, remember that the nuclear era—including nuclear weapons—is only 7-8 decades old. Like CO2, which also has a long (atmospheric) half-life, radioactive wastes from reactors will continue to accumulate, and no permanent waste disposal repositories are operational anywhere on the planet. The problem of waste disposal has been an important part of the political opposition to nuclear plants.
One proposed technical solution to this problem is 'partitioning and transmutation' (P & T). Kooyman [58] defines P & T as 'the process of separating the minor actinides from nuclear spent fuel and irradiating those using neutrons or other high-energy particles in order to trigger fission and obtain fission products with reduced half-lives'. Advantages would be reduced toxicity of wastes, and near-total burn-up of the uranium fuel. However, this complex process requires breeder reactors, and given the dwindling support for such reactors, is not likely to happen for decades, if at all.
A related problem is decommissioning reactors at the end of their operating life. The 4-unit Fukushima Daiichi nuclear power plant can serve as an example, although the melted fuel and other damage undoubtedly increased costs. The 4-unit plant cost $2.2 billion and 10 years to construct, but, according to Normile [66]: 'It's going to take roughly 30 more years and $76 billion to remove intact nuclear fuel, recover resolidified melted fuel debris, dismantle the reactors, and dispose of contaminated water'. Clearly, nuclear waste disposal and plant decommissioning are also economic problems. The radioactive nature of nuclear plant materials means that nuclear power can never really be part of the circular economy [67]; unlike former solar plants or wind turbines, the materials used for reactor construction cannot be recycled.
The climate is changing and will continue to change in likely unpredictable ways—we are moving toward a 'no-analogue' climate future [68]. Extreme weather events are rising globally [69]. Already, drought-induced shortage of cooling water has led to thermal power plants—including nuclear reactors—shutdowns [70]. On the other hand, too much water can also be a problem: Jenkins et al. [71] have argued that rising sea levels could lead to flooding for several coastal US spent fuel storage sites. Further, it was tsunami-induced flooding that severely damaged the reactors at Fukushima.
We have no real idea of what human settlement patterns will be over the next 250 years, let alone 250 millennia. Nor do we know—nor can we ever know—the future hydrological regimes of proposed waste repositories. Further, the history of temporary above-ground storage does not give confidence that an ever-rising volume of waste will be safely dealt with [72,73]. Waste disposal is a cost which nuclear operators—or their successors many centuries in the future—will inevitably attempt to minimise. The frequent failures of tailings dams in general mining should serve as a warning here [74].
Today we have many examples of near-failed states and on-going civil wars. What if nuclear power spreads to most countries, and the governance standards of a nuclear power country deteriorates? What will that mean for reactor safety, and proper and continued waste disposal and monitoring? Most discussions on nuclear safety assume that all parties are well-intentioned. Even Charles Perrow's noted book on 'Normal Accidents' [75] implicitly assumes that any likely serious nuclear events are accidents, not deliberate acts of sabotage or terrorism. Yet at least one case of a rocket attack on a nuclear installation—the Superphenix nuclear power plant in France—has been documented [76]. Even if reactors continue to be built with a concrete containment vessel, the cooling ponds, in which the intensely radioactive spent fuel rods are temporarily stored, are still vulnerable to attack. Such an attack could disperse radioactive material over a wide area, and lead to widespread panic. Additionally, as has already occurred, more countries with nuclear power programs could be expected to attempt nuclear weapons development. A number of countries have yet to ratify the UN Treaty on the non-proliferation of nuclear weapons [77].
Dittmar [36], in his paper 'The end of cheap uranium' made the case for uranium supply being a critical issue for the future of nuclear power. If nuclear power output had fulfilled its early promise, uranium reserves could indeed have been a problem today. Declining ore grades would also have increased the costs (monetary, environmental and energetic) of mining and refining uranium ores. But the stagnation in nuclear output, and the availability of enriched uranium from decommissioned nuclear warheads, have enhanced availability of fuel.
A very different problem is illustrated by the nuclear accident at Fukushima in Japan in 2011 [Kosai 78]. Not only was nuclear output lost from the damaged reactors, but many others were closed as a safety precaution, so that nuclear electricity output in Japan went from 292.4 TWh in 2010 to 162.9 TWh and then to 18.0 TWh in 2012 [6]. Although reactors normally have high availability, they are clearly vulnerable to natural and other disasters.
Markard et al. [79] concluded that the nuclear industry is in global decline, for a variety of reasons including public opposition, market liberalization and competition from renewables. Nuclear energy growth faces a combination of high costs—and financial risks—compared with other energy options, declining output in several OECD countries (and lack of interest in the nuclear option in a number of others) and long lead times for construction. It follows that nuclear power cannot be a realistic option for tackling climate change in the very limited time we have for avoiding climate catastrophe [80]. Many researchers [eg, 81-83], believe that declining costs for RE, especially wind and solar power, will render the nuclear option irrelevant.
Table 2 compares the three energy groups on the four criteria discussed in Section 4, plus two others: public health and safety and opposition to construction. The various criteria are not really independent of each other. Nuclear costs vary with the strength of opposition to nuclear power, and citizen concerns over routine emissions, reactor safety, waste repository permanence, and so on. On all criteria, all three energy groups face challenges to a greater or lesser extent. Only on the issue of weapons proliferation is nuclear energy unique. Further, the relative merits of the three energy groups could change over time, caused by technological breakthroughs, and even social and political changes. Rising future uncertainty could favour the RE sources wind and PV solar, with their very short lead times for installation.
Criterion | Nuclear | Renewable energy | Fossil fuels |
Costs | High, but difficult to separate from safety, waste disposal, and proliferation issues, etc. | Costs contested, as environmental costs not usually included. Energy storage will further raise costs. | Low at present, but will greatlyincrease if climate change costs included. |
Waste disposal | Not yet attempted at scale, but costs probably very high. | Moderate: mine tailings from ores; toxic wastes from PV manufacture. | Moderate: combustion air pollution; mine tailings disposal. |
Weapons proliferation | Weapons programs can occur under cover of civilian program. | Not a problem. | Not a problem. |
Energy security | Fuel supply a minor problem at present. | Not a problem, unless RE electricity or bioenergy pellets imported. | Can occur, as 1970s oil embargoes showed. Eventual depletion. |
Health and safety | Strongly contested: debate highly polarised. | Minor problem only. | Air pollution kills millions worldwide annually. |
Opposition | Opposition on location, environmental, health and safety grounds, etc. | Location opposition, on noise general opposition on bird/bat deaths. | Location and general environmental opposition. |
In the welcome but unlikely event that unlimited supplies of cheap RE could be rapidly implemented, the controversies would melt away. Given that the environmental costs of RE have been under-estimated—or even ignored—RE costs, technical potential, and potential for rapid displacement of FFs, have likely been greatly overestimated [18,84,85,86,87,88,89]. It is thus likely that there is no replacement for fossil fuels which is both cheap and capable of being rapidly implemented. Further, if RE is to completely replace FFs, energy storage would be needed, since the only RE sources with large technical potential are wind and solar, both intermittent sources [18]. Research on nuclear energy has pioneered the use of the full fuel cycle in analysing the impacts of nuclear energy, from the health effects of mining uranium to the environmental effects of the ultimate disposal of high-level wastes from reactors. For valid comparison, a similar approach must also be used for competing energy sources, as illustrated in Table 2. The arguments concerning nuclear vs RE are thus likely to continue. It may well be that, given the extremely uncertain times we are experiencing, reductions in energy use are the safest and fastest course of action, as are already happening in core OECD countries [6]. If so, no new OECD electrical capacity would be needed. The rise of extreme weather events, together with the social changes caused by the global pandemic, could render these reductions more feasible.
Unfortunately, the controversies might also vanish if further serious nuclear accidents or nuclear terrorism were to occur. The lessons of spectacular and widely publicised failures at Chernobyl and Fukushima resulted in major setbacks for nuclear power globally. The major failures to date have all occurred in technologically sophisticated nations. If reactor numbers increase greatly, and become widespread in countries with lower levels of either technological expertise or regulatory oversight, the chances of further major nuclear accidents will likely increase. And it is just these countries which are most in need of increased electric power capacity [90]. However, a new serious failure anywhere will further damage the prospects for nuclear power globally.
As Table 1 showed, there is no agreement on any of the key aspects of nuclear energy. Indeed, judging from the long history of these disputes, these controversies are unlikely to be satisfactorily settled any time soon. Proponents of nuclear power often point to the urgent need to respond seriously to climate change, and so argue that the current decline in nuclear power's fortunes will (or must) be revived soon. Further, the evident problems with present reactors will be solved, they argue, with new reactor types—Generation IV breeder reactors, SMRs, and even fusion reactors. Opponents can point out unresolved safety, waste disposal and weapons proliferation problems. They can also ask why these technologies have not already been adopted at scale, given their long history of development. In any case, nuclear power cannot help us solve the immediate challenge of climate change: given the long lead times for a major rise in nuclear output, it is no longer an option.
The author declares that there are no conflicts of interest related to this study.
[1] | Krymm R, Woite G (1976) Estimates of future demand for uranium and nuclear fuel cycle services. IAEA Bull 18 (5/6). |
[2] |
Lane JA (1959) Economics of nuclear power. Annu Rev Nucl Sci 9: 473-492. doi: 10.1146/annurev.ns.09.120159.002353
![]() |
[3] | Zimmermann CF, Pohl RO (1977) The potential contribution of nuclear energy to U.S. energy requirement. Energy 2: 465-471. |
[4] | Joskow PL, Baughman ML (1976) The future of the U.S. nuclear energy industry. Bell J Econ 7: 3-32. |
[5] | Moriarty P, Honnery D (2011) Rise and fall of the carbon civilisation. Springer, London, UK. |
[6] | BP (2021) BP statistical review of world energy. BP, London, UK. |
[7] | IEA (2019) Nuclear Power in a Clean Energy System. IEA Paris, France. |
[8] | Smil V (2016) "Too cheap to meter" nuclear power revisited. IEEE Spectrum. Available from: https://spectrum.ieee.org/energy/nuclear/too-cheap-to-meter-nuclear-power-revisited. |
[9] |
Bernhoft S, Sowder A, Austin R (2020) The nuclear mission in an integrated, carbon-free energy future. Responsabilité Environ 97: 107-111. doi: 10.3917/re1.097.0107
![]() |
[10] |
Froggatt A, Schneider M (2015) Nuclear power versus renewable energy: a trend analysis. Proc IEEE 103: 487-490. doi: 10.1109/JPROC.2015.2414485
![]() |
[11] | Machin A (2020) The agony of nuclear: sustaining democratic disagreement in the Anthropocene. Sustain: Sci, Practice Pol 16: 286-297. |
[12] | Moriarty P, Honnery D (2019) Sustainable energy resources: Prospects and policy. In: Rasul MG, Azad AK, Sharma SC (eds), Clean energy for sustainable development. Elsevier, London, UK. |
[13] |
Moriarty P, Honnery D (2018) Energy policy and economics under climate change. AIMS Energy 6: 272-290. doi: 10.3934/energy.2018.2.272
![]() |
[14] |
Stover D, Emanuel K (2017) A climate scientist for nuclear energy. Bull Atom Scientists 73: 7-12. doi: 10.1080/00963402.2016.1264205
![]() |
[15] |
Li J, Zhang J, Duan X (2010) Magnetic fusion development for global warming suppression. Nucl Fusion 50: 014005. doi: 10.1088/0029-5515/50/1/014005
![]() |
[16] |
Kharecha PA, Hansen JE (2013) Prevented mortality and greenhouse gas emissions from historical and projected nuclear power. Environ Sci Technol 47: 4889-4895. doi: 10.1021/es3051197
![]() |
[17] | Intergovernmental Panel on Climate Change (IPCC) (2021) Climate change 2021: The physical science basis. AR6, WG1. CUP, Cambridge UK. Available from: https://www.ipcc.ch/assessment-report/ar6/ (Also earlier reports). |
[18] | Moriarty P, Honnery D (2016) Can renewable energy power the future? Energy Pol 93: 3-7. |
[19] |
Capellán-Pérez I, de Castro C, González LJM (2019) Dynamic Energy Return on Energy Investment (EROI) and material requirements in scenarios of global transition to renewable energies. Energy Strategy Rev 26: 100399. doi: 10.1016/j.esr.2019.100399
![]() |
[20] |
Bragg-Sitton SM, Boardman R, Rabiti C, et al. (2020) Reimagining future energy systems: Overview of the US program to maximize energy utilization via integrated nuclear-renewable energy systems. Int J Energy Res 44: 8156-8169. doi: 10.1002/er.5207
![]() |
[21] | Kramer D (2018) US nuclear industry fights for survival. Phys Today 71: 26. |
[22] | Grossman L (2017) Nuclear holiday. New Sci, 20 May: 20-21. |
[23] |
Johnstone P, Sovacool BK, MacKerron G, et al. (2016) Nuclear power: serious risks. Science 354: 1112. doi: 10.1126/science.aal1777
![]() |
[24] | Lovins AB (2016) Nuclear power: deployment speed. Science 354: 1112-1113. |
[25] |
Granger Morgan M, Abdulla A, Ford MJ, et al. (2018) US nuclear power: The vanishing low-carbon wedge. PNAS 115: 7184-7189. doi: 10.1073/pnas.1804655115
![]() |
[26] |
Alonso A, Brook BW, Meneley DA, et al. (2015) Why nuclear energy is essential to reduce anthropogenic greenhouse gas emission rates. EPJ Nucl Sci Technol 1: 3. doi: 10.1051/epjn/e2015-50027-y
![]() |
[27] |
Squassoni S (2017). The incredible shrinking nuclear offset to climate change. Bull Atom Scientists 73: 17-26. doi: 10.1080/00963402.2016.1264208
![]() |
[28] | Makhijani A (2008) Nuclear isn't necessary. Nature Reports: Clim Change 2: 132-134. |
[29] |
McCombie C, Jefferson M (2016) Renewable and nuclear electricity: Comparison of environmental impacts. Energy Pol 96: 758-769. doi: 10.1016/j.enpol.2016.03.022
![]() |
[30] |
Weißbach D, Ruprecht G, Huke A, et al. (2013) Energy intensities, EROIs (energy returned on invested), and energy payback times of electricity generating power plants. Energy 52: 210-221. doi: 10.1016/j.energy.2013.01.029
![]() |
[31] |
Hall CAS, Lambert JG, Balogh SB (2014) EROI of different fuels and the implications for society. Energy Pol 64: 141-152. doi: 10.1016/j.enpol.2013.05.049
![]() |
[32] | Vine D, Juliani T (2014) Climate solutions: the role of nuclear power. Arlington, VA: Center for Climate and Energy Solutions, April: 11pp. |
[33] | Ramana MV (2021) Small modular and advanced nuclear reactors: A reality check. IEEE Access 9: 42091. |
[34] | Sekimoto H (2017) A roadmap of innovative nuclear energy system. J Phys Conf Series 799 (012001). |
[35] | Warren P, De Simone G (2014) Fuelling the future? Energy Pol 74: S5-S15. |
[36] |
Dittmar M (2013) The end of cheap uranium. Sci Total Environ 461/462: 792-798. doi: 10.1016/j.scitotenv.2013.04.035
![]() |
[37] |
Abbott A (2015) Researchers pin down risks of low-dose radiation. Nature 523: 17-18. doi: 10.1038/523017a
![]() |
[38] |
Grape S, Svä rd SJ, Hellesen C, et al. (2014) New perspectives on nuclear power—Generation IV nuclear energy systems to strengthen nuclear non-proliferation and support nuclear disarmament. Energy Pol 73: 815-819. doi: 10.1016/j.enpol.2014.06.026
![]() |
[39] | Perrow C (2011) Fukushima and the inevitability of accidents. Bull Atom Scientists 67: 44-52. |
[40] |
Rose T, Sweeting T (2016) How safe is nuclear power? A statistical study suggests less than expected. Bull Atom Scientists 72: 112-115. doi: 10.1080/00963402.2016.1145910
![]() |
[41] |
Sutou S (2020) Black rain in Hiroshima: a critique to the Life Span Study of A-bomb survivors, basis of the linear no-threshold model. Genes Environ 42: 1. doi: 10.1186/s41021-019-0141-8
![]() |
[42] | Hall H (2019) Is low-dose radiation good for you? The questionable claims for hormesis. Skeptic 24: 1. |
[43] | Takeda S, Pearson R (2018) Nuclear fusion power plants. Available from: http://dx.doi.org/10.5772/intechopen.80241. |
[44] |
Post RF (1971) Fusion power. PNAS 68: 1931-1937. doi: 10.1073/pnas.68.8.1931
![]() |
[45] | Moriarty P, Honnery D (2007) Intermittent renewable energy: The only future source of hydrogen? Int J Hydrog Energy 32: 1616-1624. |
[46] | Kembleton R (2019) Nuclear fusion: What of the future? In Letcher T (ed), Managing global warming: An interface of technology and human issues. Academic Press, London, UK., pp.199-220. |
[47] | Jassby D (2018) ITER is a showcase... for the drawbacks of fusion energy. Available from: https://sites.nationalacademies.org/cs/groups/bpasite/documents/webpage/bpa_184876.pdf. |
[48] | Wikipedia (2021) Generation IV reactor. Available from: https://en.wikipedia.org/wiki/Generation_IV_reactor. |
[49] |
D'Auria F, Debrecin N, Glaeser H (2019) The technological challenge for current generation nuclear reactors. J Nucl Energy Technol (NUCET) 5: 183-199. doi: 10.3897/nucet.5.38117
![]() |
[50] |
Sovacool BK, Ramana MV (2015) Back to the future: small modular reactors, nuclear fantasies, and symbolic convergence. Sci, Technol, Human Values 40: 96-125. doi: 10.1177/0162243914542350
![]() |
[51] |
Mignacca B, Locatelli G, Sainati T (2020) Deeds not words: Barriers and remedies for Small Modular nuclear Reactors. Energy 206: 118137. doi: 10.1016/j.energy.2020.118137
![]() |
[52] |
Hussein EMA (2020) Emerging small modular nuclear power reactors: A critical review. Phys Open 5: 100038. doi: 10.1016/j.physo.2020.100038
![]() |
[53] | International Atomic Energy Agency (IAEA) (2018) Experience in modelling nuclear energy systems with MESSAGE: Country case studies. IAEA-TECDOC-1837. Available from: https://www-pub.iaea.org/MTCD/Publications/PDF/TE-1837web.pdf. |
[54] | Buongiorno J, Carmichael B, Dunkin B, et al. (2021) Can nuclear batteries be economically competitive in large markets? Energies 4385. |
[55] |
Testoni R, Bersano A, Segantin S (2021) Review of nuclear microreactors: Status, potentialities and challenges. Progr Nucl Energy 138: 103822. doi: 10.1016/j.pnucene.2021.103822
![]() |
[56] | Weinberg AM, Hammond RP (1973) Limits to the use of energy. Am Sci 58: 412-418. |
[57] |
Locatelli G, Mancini M, Todeschini N (2013) Generation IV nuclear reactors: Current status and future prospects. Energy Pol 61: 1503-1520. doi: 10.1016/j.enpol.2013.06.101
![]() |
[58] |
Kooyman T (2021) Current state of partitioning and transmutation studies for advanced nuclear fuel cycles. Annals Nucl Energy 157: 108239. doi: 10.1016/j.anucene.2021.108239
![]() |
[59] | Hoegh-Guldberg O, Jacob D, Taylor M, et al. (2019) The human imperative of stabilizing global climate change at 1.5 ℃. Science 365: 1263. |
[60] |
Hirsch RL (2017) Necessary and sufficient conditions for practical fusion power. Phys Today 70: 11-13. doi: 10.1063/PT.3.3746
![]() |
[61] | Hassanein A, Sizyuk V (2021) Potential design problems for ITER fusion device. Sci Reports 11: 2069. |
[62] | Coady D, Parry I, Louis Sears L, et al. (2017) How Large Are Global Fossil Fuel Subsidies? World Dev 91: 11-27. |
[63] |
Sovacool BK, Hess DJ, Amir S, et al. (2020) Sociotechnical agendas: Reviewing future directions for energy and climate research. Energy Res Soc Sci 70: 101617. doi: 10.1016/j.erss.2020.101617
![]() |
[64] |
Wealer B, Bauer S, Hirschhausen Cv, et al. (2021) Investing into third generation nuclear power plants - Review of recent trends and analysis of future investments using Monte Carlo Simulation. Renewable Sustainable Energy Rev 143: 110836. doi: 10.1016/j.rser.2021.110836
![]() |
[65] | Anderson M (2020) limited progress for U.S. nuclear. IEEE Spectrum June: 6-7. |
[66] |
Normille D (2021) Why cleaning up Fukushima's damaged reactors will take another 30 years. Science 371: 983. doi: 10.1126/science.371.6533.983
![]() |
[67] |
Velenturf APM, Purnell P (2021) Principles for a sustainable circular economy. Sustain Prod Consumption 27: 1437-1457. doi: 10.1016/j.spc.2021.02.018
![]() |
[68] |
Fitzpatrick MC, Blois JL, Williams JW, et al. (2018) How will climate novelty influence ecological forecasts? Using the Quaternary to assess future reliability. Glob Change Biol 24: 3575-3586. doi: 10.1111/gcb.14138
![]() |
[69] | Lange S, Volkholz J, Geiger T, et al. (2020) Projecting exposure to extreme climate impact events across six event categories and three spatial scales. Earth's Future 11: e2020EF001616. |
[70] |
Büntgen U, Urban O, Krusic PJ, et al. (2021) Recent European drought extremes beyond Common Era background variability. Nature Geosci 14: 190-196. doi: 10.1038/s41561-021-00698-0
![]() |
[71] | Jenkins LS, Alvarez R, Jordaan SM (2020) Unmanaged climate risks to spent fuel from U.S. nuclear power plants: The case of sea-level rise. Energy Pol 137: 111106. |
[72] | MacKerron G (2019) Future prospects on coping with nuclear waste. In: Haas R, Mez L, Ajanovic A (Eds) The technological and economic future of nuclear power. Springer VS. Available from: https://doi.org/10.1007/978-3-658-25987-7_1. |
[73] | Bowrey B (2020) Nuclear waste and society: A historiographic review and analysis of two approaches. Intersect 14: 1-16. |
[74] | Cornwall W (2020) A dam big problem. Science 369: 907-909. |
[75] | Perrow C (1999) Normal accidents: Living with high risk technologies (Updated Edition). Princeton Univ Press, Princeton, NJ, USA. |
[76] | Wikipedia (2021) Superphenix. Available from: https://en.wikipedia.org/wiki/Superphenix. |
[77] | United Nations (UN) (2021) Treaty on the non-proliferation of nuclear weapons (NPT). Available from: https://www.un.org/disarmament/wmd/nuclear/npt/. |
[78] |
Kosai S, Unesaki H (2017) Quantitative analysis on the impact of nuclear energy supply disruption on electricity supply security. Appl Energy 208: 1198-1207. doi: 10.1016/j.apenergy.2017.09.033
![]() |
[79] |
Markard J, Bento N, Kittner N, et al. (2020) Destined for decline? Examining nuclear energy from a technological innovation systems perspective. Energy Res Soc Sci 67: 101512. doi: 10.1016/j.erss.2020.101512
![]() |
[80] | Ripple WJ, Wolf C, Newsome T, et al. (2019) World scientists' warning of a climate emergency. BioSci: 1-5. |
[81] |
Jacobson MZ (2017) Roadmaps to transition countries to 100% clean, renewable energy for all purposes to curtail global warming, air pollution, and energy risk. Earth's Future 5: 948-952. doi: 10.1002/2017EF000672
![]() |
[82] | Davis SJ, Lewis NS, Shaner M, et al. (2018) Net-zero emissions energy systems. Science 360: eaas9793. |
[83] | Kammen DM (2020) Over the hump: Have we reached the peak of carbon emissions? Bull Atom Sci 76: 256-262. |
[84] |
Smil V (2018) It'll be harder than we thought to get the carbon out. IEEE Spectr 55: 72-75. doi: 10.1109/MSPEC.2018.8362233
![]() |
[85] |
Moriarty P, Honnery D (2020) Feasibility of a 100% global renewable energy system. Energies 13: 5543. doi: 10.3390/en13215543
![]() |
[86] |
Heard B, Brook B, Wigley T, et al. (2017) Burden of proof: A comprehensive review of the feasibility of 100% renewable-electricity systems. Renewable Sustainable Energy Rev 76: 1122-1133. doi: 10.1016/j.rser.2017.03.114
![]() |
[87] |
Moriarty P, Wang SJ (2015) Assessing global renewable energy forecasts. Energy Proc 75: 2523-2528. doi: 10.1016/j.egypro.2015.07.256
![]() |
[88] |
Nieto J, Carpintero O, Miguel LJ, et al. (2020) Macroeconomic modelling under energy constraints: Global low carbon transition scenarios. Energy Pol 137: 111090. doi: 10.1016/j.enpol.2019.111090
![]() |
[89] |
Moriarty P, Honnery D (2021) The limits of renewable energy. AIMS Energy 9: 812-829. doi: 10.3934/energy.2021037
![]() |
[90] | International Energy Agency (IEA) (2020) Key world energy statistics 2020. Paris, IEA/OECD. |
1. | Patrick Moriarty, Damon Honnery, When will the hydrogen economy arrive?, 2022, 10, 2333-8334, 1100, 10.3934/energy.2022052 | |
2. | Jinming Xue, Zongbo Xie, Bo Wang, Ye'an Zhu, Zhiwen Wu, Yidan Nie, Zhanggao Le, Enhanced visible-light photocatalytic performance by PPy/CN composites for reduction of UO22+, 2022, 315, 00224596, 123440, 10.1016/j.jssc.2022.123440 | |
3. | Patrick Moriarty, Damon Honnery, 2022, Chapter 2, 978-981-19-0766-1, 15, 10.1007/978-981-19-0767-8_2 | |
4. | Jan Wrana, Wojciech Struzik, Bartłomiej Kwiatkowski, Piotr Gleń, Release of Energy from Groundwater/with Reduction in CO2 Emissions of More Than 50% from HVAC in the Extension and Revitalization of the Former Palace of the Sobieski Family in Lublin, 2022, 15, 1996-1073, 6627, 10.3390/en15186627 | |
5. | Zhanggao Le, Jinming Xue, Bo Wang, Ye’an Zhu, Zhiwen Wu, Yidan Nie, Zongbo Xie, MIL-100(Fe)/g-C3N4 composites with enhanced photocatalytic activity for UO22+ reduction under visible light, 2022, 22, 1474-9092, 59, 10.1007/s43630-022-00298-w | |
6. | Patrick Moriarty, Damon Honnery, Review: Renewable Energy in an Increasingly Uncertain Future, 2022, 13, 2076-3417, 388, 10.3390/app13010388 | |
7. | Patrick Moriarty, Electric vehicles can have only a minor role in reducing transport's energy and environmental challenges, 2022, 10, 2333-8334, 131, 10.3934/energy.2022008 | |
8. | Patrick Moriarty, Damon Honnery, Renewable Energy and Energy Reductions or Solar Geoengineering for Climate Change Mitigation?, 2022, 15, 1996-1073, 7315, 10.3390/en15197315 | |
9. | Peng Shen, Runfeng Zhang, Zhigang Wang, Jiangyi Chen, Kinetic Modeling and Analysis of Fuel Element Pneumatic Lifting System in Pebble Bed Reactors, 2023, 13, 2076-3417, 1486, 10.3390/app13031486 | |
10. | Jinming Xue, Zong-Bo Xie, Bo Wang, Ye’an Zhu, Zhiwen Wu, Yidan Nie, Zhanggao Le, Enhanced Visible-Light Photocatalytic Performance by Ppy/Cn Composites for Reduction of Uo22+, 2022, 1556-5068, 10.2139/ssrn.4111177 | |
11. | Oscar Eliezer Mendoza-De Los Santos, Intellectual Virtues and Scientific Endeavor: A Reflection on the Commitments Inherent in Generating and Possessing Knowledge, 2023, 43, 0270-4676, 18, 10.1177/02704676231171318 | |
12. | Patrick Moriarty, 2023, Chapter 15, 978-3-031-38505-6, 389, 10.1007/978-3-031-38506-3_15 | |
13. | Feng Gong, Jiaming Song, Haotian Chen, Hao Li, Runnan Huang, Yuhang Jing, Peng Yang, Junjie Feng, Rui Xiao, A fibrous hydroelectric generator derived from eco-friendly sodium alginate for low-grade energy harvesting, 2024, 18, 2095-1701, 474, 10.1007/s11708-024-0930-z | |
14. | Joel Augusto Moura Porto, Lander de Jesus Alves, Fábio Carvalho Nunes, Eduardo Gross, Hector Hugo Silva Medrado, Josilene da Silva Rocha, Marcelo Machado Viana, Ana Paula de Carvalho Teixeira, Majeti Narasimha Vara Prasad, 2024, 9780443136078, 97, 10.1016/B978-0-443-13607-8.00009-2 | |
15. | Patrick Moriarty, Damon Honnery, Review: The Energy Implications of Averting Climate Change Catastrophe, 2023, 16, 1996-1073, 6178, 10.3390/en16176178 | |
16. | Patrick Moriarty, Damon Honnery, 2025, 9781394204380, 73, 10.1002/9781394204847.ch5 | |
17. | Jialin Song, Haoyi Zhang, Yanming Zhang, Zhongjiao Ma, Mingfei He, Research progress on industrial waste heat recycling and seasonal energy storage, 2025, 13, 2333-8334, 147, 10.3934/energy.2025006 |
Nuclear Energy controversy | 'Optimistic' papers | 'Pessimistic' papers |
Nuclear power's future | [1,2,3,4] | [13,21,22,23,24,25] |
Nuclear energy essential for climate change mitigation | [14,15,16,17,26] | [25,27,28] |
Energy return on energy invested (EROI) for nuclear energy | [29,30] | [5,31] |
Future costs for nuclear energy | [32] | [10,33] |
Size and cost of uranium reserves | [34,35] | [36] |
Environmental benefits of nuclear power | [16] | [37] |
Dangers of nuclear weapons proliferation | [38] | [5,23] |
Risk from serious nuclear accidents | [34] | [39,40] |
Health effects of low-level radiation | [41] | [42] |
Successful introduction of fusion power | [15,43,44] | [45,46,47] |
Criterion | Nuclear | Renewable energy | Fossil fuels |
Costs | High, but difficult to separate from safety, waste disposal, and proliferation issues, etc. | Costs contested, as environmental costs not usually included. Energy storage will further raise costs. | Low at present, but will greatlyincrease if climate change costs included. |
Waste disposal | Not yet attempted at scale, but costs probably very high. | Moderate: mine tailings from ores; toxic wastes from PV manufacture. | Moderate: combustion air pollution; mine tailings disposal. |
Weapons proliferation | Weapons programs can occur under cover of civilian program. | Not a problem. | Not a problem. |
Energy security | Fuel supply a minor problem at present. | Not a problem, unless RE electricity or bioenergy pellets imported. | Can occur, as 1970s oil embargoes showed. Eventual depletion. |
Health and safety | Strongly contested: debate highly polarised. | Minor problem only. | Air pollution kills millions worldwide annually. |
Opposition | Opposition on location, environmental, health and safety grounds, etc. | Location opposition, on noise general opposition on bird/bat deaths. | Location and general environmental opposition. |
Nuclear Energy controversy | 'Optimistic' papers | 'Pessimistic' papers |
Nuclear power's future | [1,2,3,4] | [13,21,22,23,24,25] |
Nuclear energy essential for climate change mitigation | [14,15,16,17,26] | [25,27,28] |
Energy return on energy invested (EROI) for nuclear energy | [29,30] | [5,31] |
Future costs for nuclear energy | [32] | [10,33] |
Size and cost of uranium reserves | [34,35] | [36] |
Environmental benefits of nuclear power | [16] | [37] |
Dangers of nuclear weapons proliferation | [38] | [5,23] |
Risk from serious nuclear accidents | [34] | [39,40] |
Health effects of low-level radiation | [41] | [42] |
Successful introduction of fusion power | [15,43,44] | [45,46,47] |
Criterion | Nuclear | Renewable energy | Fossil fuels |
Costs | High, but difficult to separate from safety, waste disposal, and proliferation issues, etc. | Costs contested, as environmental costs not usually included. Energy storage will further raise costs. | Low at present, but will greatlyincrease if climate change costs included. |
Waste disposal | Not yet attempted at scale, but costs probably very high. | Moderate: mine tailings from ores; toxic wastes from PV manufacture. | Moderate: combustion air pollution; mine tailings disposal. |
Weapons proliferation | Weapons programs can occur under cover of civilian program. | Not a problem. | Not a problem. |
Energy security | Fuel supply a minor problem at present. | Not a problem, unless RE electricity or bioenergy pellets imported. | Can occur, as 1970s oil embargoes showed. Eventual depletion. |
Health and safety | Strongly contested: debate highly polarised. | Minor problem only. | Air pollution kills millions worldwide annually. |
Opposition | Opposition on location, environmental, health and safety grounds, etc. | Location opposition, on noise general opposition on bird/bat deaths. | Location and general environmental opposition. |