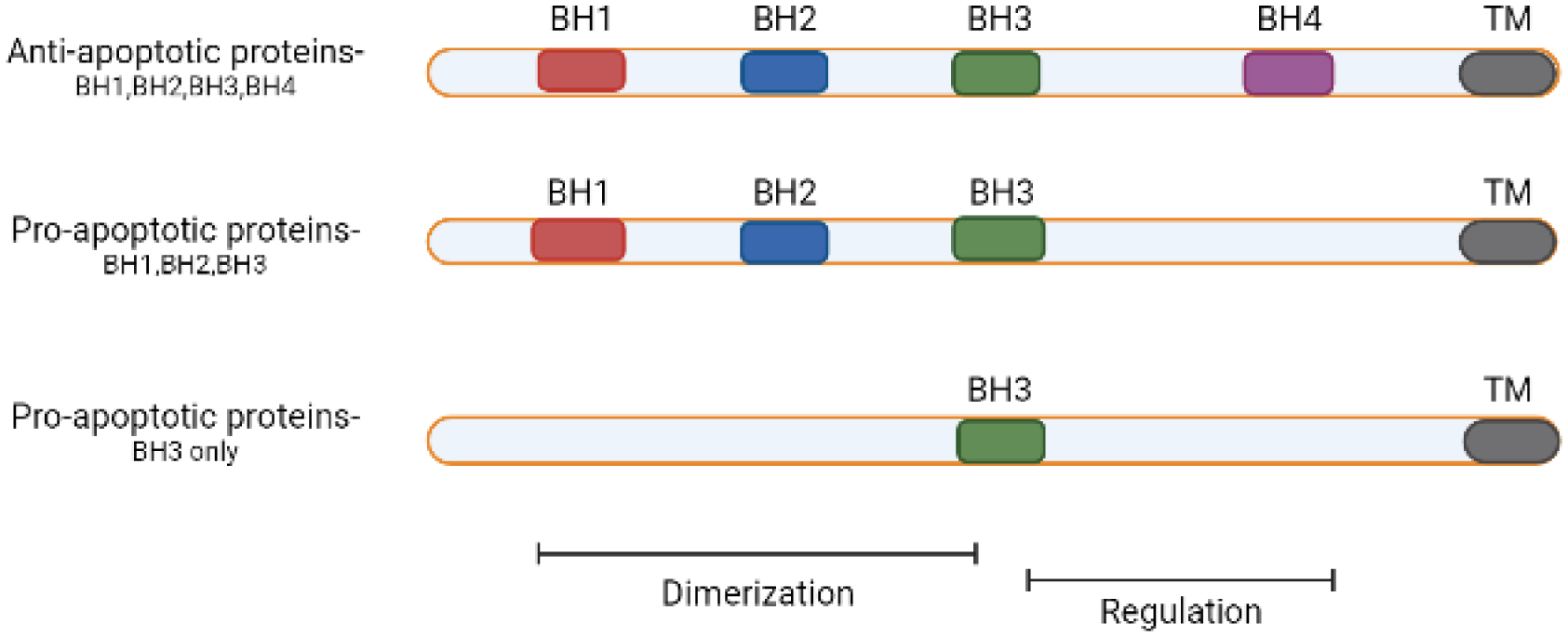
Breast cancer, a prominent form of cancer in women, arises from the inner lining of mammary glands, ducts, and lobules. With an approximate prevalence rate of 1 in 8 women, the standard treatment methods for this condition include the surgical excision of afflicted tissues, chemotherapy, radiation, and hormone therapy. The BCL-2 gene, also known as the B cell lymphoma gene, prevents apoptosis in eukaryotic cells. It is commonly found to be excessively active in many types of malignancies, such as leukemia, carcinomas, and breast cancer. The excessive expression of this gene has a role in the advancement of cancer by inhibiting apoptosis. Recent research emphasizes the function of microRNAs (miRs) in regulating the expression of BCL-2. These miRs can either decrease or increase the activity of specific genes involved in programmed cell death, thus making them potential targets for therapeutic interventions. This review explicitly examines the regulatory impacts of several miRs on BCL-2, thereby investigating their ability to trigger apoptosis and function as targeted treatments for breast cancer. By comprehending the complex interplay between miRs and BCL-2, it is possible to devise novel therapeutic approaches that can augment the efficacy of breast cancer treatments, thus eventually enhancing patient outcomes.
Citation: Kannan Mayuri, Sundaram Vickram, Thirunavukarasou Anand, Konda Mani Saravanan. MicroRNA-mediated regulation of BCL-2 in breast cancer[J]. AIMS Molecular Science, 2025, 12(1): 32-48. doi: 10.3934/molsci.2025003
[1] | Xin Nian, Yasuhiro Nagai, Cameron Jeffers, Kara N. Maxwell, Hongtao Zhang . Dietary influence on estrogens and cytokines in breast cancer. AIMS Molecular Science, 2017, 4(3): 252-270. doi: 10.3934/molsci.2017.3.252 |
[2] | Irene Mwongeli Waita, Atunga Nyachieo, Daniel Chai, Samson Muuo, Naomi Maina, Daniel Kariuki, Cleophas M. Kyama . Differential expression and functional analysis of micro RNAs in Papio anubis induced with endometriosis for early detection of the disease. AIMS Molecular Science, 2020, 7(4): 305-327. doi: 10.3934/molsci.2020015 |
[3] | Abdulrahman Mahmoud Dogara, Ateeq Ahmed Al-Zahrani, Sarwan W. Bradosty, Saber W. Hamad, Aisha Abdullahi Mahmud, Hussain D. Almalki, Mustapha Abdullahi, Abubakar Abdullahi Lema, Hasan Nudin Nur Fatihah . In-vitro biological activity and in-silico studies of some volatile phytochemicals from the ethanol extract of Eugenia uniflora. AIMS Molecular Science, 2024, 11(3): 303-321. doi: 10.3934/molsci.2024018 |
[4] | Sujit Nair . Current insights into the molecular systems pharmacology of lncRNA-miRNA regulatory interactions and implications in cancer translational medicine. AIMS Molecular Science, 2016, 3(2): 104-124. doi: 10.3934/molsci.2016.2.104 |
[5] | Chisato Kinoshita, Koji Aoyama, Toshio Nakaki . microRNA as a new agent for regulating neuronal glutathione synthesis and metabolism. AIMS Molecular Science, 2015, 2(2): 124-143. doi: 10.3934/molsci.2015.2.124 |
[6] | Seidu A. Richard . High-mobility group box 1 is a promising diagnostic and therapeutic monitoring biomarker in Cancers: A review. AIMS Molecular Science, 2018, 5(4): 183-241. doi: 10.3934/molsci.2018.4.183 |
[7] | Karen E. Livermore, Jennifer Munkley, David J. Elliott . Androgen receptor and prostate cancer. AIMS Molecular Science, 2016, 3(2): 280-299. doi: 10.3934/molsci.2016.2.280 |
[8] | Naba Hasan, Waleem Ahmad, Feroz Alam, Mahboob Hasan . Ferroptosis-molecular mechanisms and newer insights into some diseases. AIMS Molecular Science, 2023, 10(1): 22-36. doi: 10.3934/molsci.2023003 |
[9] | Jin-Yih Low, Helen D. Nicholson . Investigating the role of caveolin-2 in prostate cancer cell line. AIMS Molecular Science, 2017, 4(1): 82-88. doi: 10.3934/molsci.2017.1.82 |
[10] | Simone Leggeri, Navid Sobhani . Single nucleotide polymorphisms Rs1045642 C>T genetic alteration in ATP Binding Cassette Subfamily B Member 1 role in increasing everolimus toxicity in metastatic breast cancer. AIMS Molecular Science, 2020, 7(1): 1-11. doi: 10.3934/molsci.2020001 |
Breast cancer, a prominent form of cancer in women, arises from the inner lining of mammary glands, ducts, and lobules. With an approximate prevalence rate of 1 in 8 women, the standard treatment methods for this condition include the surgical excision of afflicted tissues, chemotherapy, radiation, and hormone therapy. The BCL-2 gene, also known as the B cell lymphoma gene, prevents apoptosis in eukaryotic cells. It is commonly found to be excessively active in many types of malignancies, such as leukemia, carcinomas, and breast cancer. The excessive expression of this gene has a role in the advancement of cancer by inhibiting apoptosis. Recent research emphasizes the function of microRNAs (miRs) in regulating the expression of BCL-2. These miRs can either decrease or increase the activity of specific genes involved in programmed cell death, thus making them potential targets for therapeutic interventions. This review explicitly examines the regulatory impacts of several miRs on BCL-2, thereby investigating their ability to trigger apoptosis and function as targeted treatments for breast cancer. By comprehending the complex interplay between miRs and BCL-2, it is possible to devise novel therapeutic approaches that can augment the efficacy of breast cancer treatments, thus eventually enhancing patient outcomes.
Breast cancer (BC) is a complex and diverse condition marked by notable differences among those affected, thus posing a considerable obstacle in the development of successful therapies [1],[2]. Notwithstanding the intricacy involved, improvements in diagnostic technologies have decreased the mortality rates related to BC [3],[4]. Further advancements in the timely identification, assessment, and mitigation of BC are essential to enhance the survival rates [5]–[7]. BC is mainly caused by unregulated cell growth in the inner lining of mammary glands and ducts [8]. The uncontrolled growth of cells is frequently aided by the suppression of apoptosis, which is a biological process controlled by the BCL-2 protein family [9]. Conventional BC therapies include hormone therapy, surgical procedures, chemotherapy, and radiation therapy [10]. Although these treatments can yield positive results, they frequently come with notable adverse effects such as post-operative infections, sensory impairments, toxicities, and drug resistance [11]. The intricate origin of BC encompasses various phases, including tumor initiation, development, metastasis, invasion, angiogenesis, and recurrence [12]. Disturbances in cellular and molecular signaling networks mark each stage. These characteristics emphasize the necessity for more focused and less harmful therapeutic approaches [13].
Among the several genetic variables implicated in BC, the BCL-2 gene emerges as a pivotal controller of programmed cell death (apoptosis) [14]. BCL-2, a proto-oncogene, acts as an inhibitor of cell death and is a member of a protein family with essential responsibilities in regulating programmed cell death [15]. The change in a chromosomal location, t(14;18), which is found in certain types of cancer of the lymphatic system, such as non-Hodgkin's lymphoma, results in the over-production of the BCL-2 protein that aids cancer growth [16]. Additionally, this upregulation has been detected in many solid malignancies, including BC [17]. In addition to its involvement in cancer, the BCL-2 family of proteins regulates apoptosis, tissue formation, and immunological responses to pathogens [18]. Mammals possess more than twelve fundamental proteins in the BCL-2 family, which exhibit structural resemblances to BCL-2 and play several crucial functions in biological processes, such as controlling programmed cell death [19].
Recent studies have emphasized the substantial influence of microRNAs (miRs) in the control of BC [20]–[22]. miRs are tiny, single-stranded RNA molecules that indirectly affect physiological activities and directly regulate gene transcription [23]. miRs can either enhance or inhibit gene expression depending on their biological roles, thereby functioning as either tumor suppressor miRNAs (tsmiRs) or oncogenic miRNAs (oncomiRs) [24]. The dysregulated expression of miRs has been detected at several phases of BC, ranging from the early stages of tumor development to cell death [25]. By influencing critical processes such as cell proliferation, apoptosis, and cancer cell metastasis, TsmiRs and oncomiRs are essential in BC control [26].
Recent research suggests that miRs have a role in regulating the expression of BCL-2, thus indicating that manipulating miRs could potentially increase apoptosis in BC cells [27],[28]. This offers a hopeful treatment approach, as miRs that decrease the expression of BCL-2 can counteract one of the main ways BC cells avoid programmed cell death [29]. By prioritizing the regulatory correlation between miRs and BCL-2, it is possible to devise novel therapeutic approaches that are more specific and potentially less harmful than conventional treatments [30]. Implementing these measures can greatly enhance the treatment outcomes for patients with BC. This review emphasizes the possibility of miR-based therapeutics as a new and successful to treat BC by studying the different miRs that control BCL-2 expression and their ability to trigger apoptosis. By gaining a more profound comprehension of the miR-BCL-2 regulatory axis, we can devise inventive therapeutic approaches that augment the effectiveness of BC treatments and eventually boost the patient outcomes.
The BCL-2 protein family is crucial in controlling apoptosis, which involves maintaining a careful balance between cell survival and programmed cell death (PCD) [31]. This family includes substances that prevent and promote apoptosis, thus demonstrating its essential role in maintaining cellular balance [32]. BCL-2 proteins, which vary in size from 20 to 37 kDa, have substantial similarities in their amino acid sequences [33]. They are categorized into three primary classifications, depending on their functions in apoptosis. Antiapoptotic proteins can prevent apoptosis and have BCL-2 homology domains BH-1, BH-2, BH-3, and BH-4 [34]; notable members of this class include BCL-2, BCL-W, MCL1, BCL-XL, BCL-2A1, and BCL-B. Pro-apoptotic proteins promote apoptosis and typically possess three BH domains [35]; the proteins BAX, BAK, and BOK are noteworthy members of this category. The pro-apoptotic class of proteins is characterized by its diversity and consists solely of proteins with the BH-3 domain (i.e., BH3-only proteins) (Figure 1); some examples of proteins in this class include BIK, BID, BAD, BIM, BMF, NOXA, HRK, and PUMA [36],[37]. These proteins can attach to and control antiapoptotic BCL-2 proteins, resulting in the initiation of apoptosis.
BCL-2 proteins primarily function on the outer membranes of the mitochondria and the endoplasmic reticulum [38]. Their location and activity are facilitated by a hydrophobic anchor located near the C-terminal of the BCL-2 homologous region. BCL-2 proteins typically adopt a structural conformation characterized by α-helical hairpins and tend to form heterodimers [39]. Heterodimerization occurs when the BH3 region of a pro-apoptotic protein binds to the hydrophobic groove of an antiapoptotic protein, which contains BH1, BH2, and BH3 domains. The BH3 domain plays a crucial role in promoting apoptosis, whereas antiapoptotic proteins need numerous BH domains to successfully carry out their action [40]. Mitochondrial Outer Membrane Permeabilization (MOMP) is a crucial mechanism that regulates apoptosis by BCL-2 proteins [41]. MOMP causes the liberation of apoptotic components, including cytochrome c and Apoptosis Promoting Factor (APF), from the mitochondria into the cytoplasm [42]. The caspase cascade, which causes cells to die, starts with this occurrence. Antiapoptotic proteins are promoted by pro-apoptotic proteins, such as BAX and BAK [43]. In addition to inhibiting antiapoptotic proteins, BH3-only proteins can enhance the activation of BAX and BAK. This dual action ultimately leads to the promotion of apoptosis [44].
miRs are small RNA molecules which naturally occur within an organism and have a single strand [23]. miRs are synthesized from double-stranded DNA by RNA polymerases II and III, thus forming primary miRNAs (pri-miRs) [45]. The enzyme Drosha, which belongs to the RNA POL III family, processes these pri-miRs that produce precursor miRNAs (pre-miRs) in the nucleus [46]. Pre-miRs are subsequently transported to the cytoplasm by Exportin-5, which is a protein that relies on GTP for its function [47]. Within the cytoplasm, the enzyme Dicer, which belongs to the RNA POL III family, trims pre-miRs to produce mature miRs [48]. These miRs are generally around 20–22 nucleotides long. The mature miRs are integrated into the RNA-induced silencing complex (RISC), which aids in breaking target miRs, thus suppressing gene expression [49]. When miRs attach to the 3′ untranslated region and 5′ untranslated region of target miRs, it leads to the inhibition of translation, which effectively inhibits gene expression. miRs participate in diverse biological processes, such as cell proliferation, cellular specialization, morphogenesis, and apoptosis [50]. Figure 2 demonstrates that miRs selectively cut target-specific mRNAs to prevent non-specific binding and control these biological processes. Significantly, a solitary miR can control numerous genes, while a solitary gene can be subjected to the influence of multiple miRs.
Recent research has extensively concentrated on identifying certain miRs linked to cancer apoptosis and their corresponding target genes [51]. Tumor suppressor miRs, frequently downregulated in cancer, are crucial in impeding tumor growth by negatively influencing oncogenes or genes that control cell differentiation and apoptosis [52]. Tumor-suppressing miRs can be downregulated due to deletions, epigenetic silencing, or a lower expression of transcription factors. Due to their crucial involvement in multiple biological processes, changes in miR expression are associated with a wide range of human illnesses, including cancer [53]. Research has demonstrated that malignant tumors and tumor cell lines display extensive and abnormal miR expression compared to normal tissues [54]. During carcinogenesis, miRs play a crucial role in regulating apoptosis. Cancer cells can modify miRs to govern cell survival during cancer development [55].
The intrinsic pathway is a well-researched mechanism of apoptosis [56]. In this process, cytochrome c is released from the mitochondria and moves into the cytoplasm. Once in the cytoplasm, it triggers the activation of caspases by interacting with the apoptotic peptidase activating factor-1 [57]. Hence, miRNAs that govern these molecules can profoundly impact the efficacy of anticancer medications [58]. A comprehensive understanding of these regulatory systems is crucial to identify and develop effective treatment targets for BC [59].
Many researchers have aimed to find miRs linked to cancer apoptosis and their corresponding target genes [60]. Table 1 provides a comprehensive list of several miRs that control the BCL-2 family, emphasizing their potential as targets for therapeutic interventions in BC. By manipulating the expression and function of these miRs, it would be feasible to augment the efficacy of BC treatments, enhance the patient outcomes, and devise novel therapeutic approaches. The comprehensive comprehension of miR control of BCL-2 and its family members offers vital clues that underlie BC and presents new possibilities for targeted therapies [44].
Target gene | miR | Regulation | Reference |
BCL-2 | miR-7 | Inhibits target BCL-2 gene directly | [61] |
BCL-2 | miR-15a/16 | Inhibits target BCL-2 gene directly | [62] |
BCL-2 | miR-21 | Activates target BCL-2 gene directly | [63] |
BCL-2 | miR-24–2 | Inhibits target BCL-2gene directly | [64] |
BIM | miR-24-3p | Inhibits target BIM gene directly | [65] |
BCL-2 | miR-31 | Inhibits target BCL-2 gene directly | [66] |
BCL-2 | miR-34a | Inhibits target BCL-2 gene directly | [67] |
BCL-2 BAX |
miR-93 | Inhibits target BCL-2 gene directly Inhibits target BAX gene directly |
[68] |
BCL-2 | miR-106 | Activates target BCL-2 gene directly | [69] |
BCL-2 | miR-122-5p | Inhibits target BCL-2gene directly | [70] |
BCL-2 BAK1 |
miR-125b | Inhibits target BCL-2 gene directly Inhibits target BAK1 gene directly |
[71],[72] |
BCL-2 | miR-134 | Inhibits target BCL-2 gene directly | [73] |
BAX | miR-149-5p | Inhibits target BAX gene directly | [74] |
BIM | miR-181b | Inhibits target BIM gene directly | [75] |
BAD | miR-192-5b | Inhibits target BAD gene directly Inhibits target BCL-2 gene indirectly |
[76] |
BCL-2 | miR-195 | Inhibits target BCL-2 gene directly | [77] |
BCL-XL | miR-203a-3p miR-203b-3p |
Inhibits target BCL-XL gene directly Inhibits target BCL-XL gene directly |
[78] |
BIM BAX BAK | miR-221 | Inhibits target BIM gene directly | [79] |
BIM | miR-222 | Inhibits target BIM gene directly | [80] |
BCL-2 | miR-451 | Inhibits target BCL-2 gene directly | [81] |
BCL-2 | miR-489 | Inhibits target BCL-2 gene directly | [82] |
BAX* | miR-663b | Activates target BCL-2 gene directly | [83] |
The table provides a concise overview of different miRs that control the expression of BCL-2 and other genes involved in programmed cell death in BC. Several miRs, including miR-7, miR-15a/16, miR-24–2, miR-31, miR-34a, miR-93, miR-122-5p, miR-125b, miR-134, miR-195, miR-451, and miR-489, directly hinder the expression of the BCL-2 gene, which is known to facilitate programmed cell death, and hence function as tumor suppressors. Additional miRNAs, such as miR-21 and miR-106, stimulate the activation of BCL-2, which hinders programmed cell death and potentially enhances the ability of cancer cells to survive. In addition, miRs such as miR-93, miR-125b, and miR-192-5b can target both pro-apoptotic genes BAX and BAK1, thus suggesting the existence of an intricate regulatory network. miR-149-5p, miR-181b, miR-221, and miR-222 precisely target genes such as BIM and BAD, thereby exerting an additional influence on the pathways that regulate cell death (apoptosis). Remarkably, miR-663b stimulates the activation of BCL-2, which contrasts with its typical suppression by other miRs. The varied regulatory impacts of these miRs on BCL-2 and the associated genes emphasize their potential as therapeutic targets to manipulate apoptosis in breast cancer, thus providing opportunities to enhance cancer cell death and overcome resistance to traditional treatments.
Numerous studies have shown that miRs significantly affect the upregulation or downregulation of BCL-2 family proteins, which is pivotal in regulating apoptosis in breast cancer. Several principal miR species have been identified that interact with BCL-2 to influence tumor cell viability directly. For example, miR-34a is a recognized tumor suppressor often downregulated in breast cancer and has been shown to post-translationally control BCL-2 by binding to its 3′ UTR [84]. This interaction facilitates the degradation of BCL-2, thereby initiating apoptotic pathways that result in the death of tumor cells. Similarly, miR-15a/16, which is often deleted or downregulated in several neoplasms, including breast cancer, diminishes BCL-2 levels and promotes apoptosis [85]. Conversely, some miRs, such as miR-21, have been identified to elevate BCL-2 levels, thus enhancing chemoresistance by facilitating the survival of cancer cells [86]. The pro-survival effect is particularly significant in breast cancer, where miR-21 is overexpressed and linked to an unfavorable prognosis. Furthermore, it has been shown that miR-125b directly interacts with BCL-2, thereby altering the apoptotic threshold to promote cancer cell survival [72]. These miRs target BCL-2 within a complex signaling network that, in addition to influencing the mitochondrial integrity, also governs essential apoptotic events, including the release of cytochrome c and the activation of caspases. The deregulation of these miRs in breast cancer may indicate their potential as biomarkers for early diagnoses and therapeutic approaches [87]. Clarifying the complex mechanisms via which miRs regulate BCL-2 and its associated family members is crucial to develop novel miRNA-based therapeutic approaches aimed at inducing tumor cell apoptosis and surmounting resistance to standard treatments.
The fundamental characteristics of miR activities suggest a complex interaction with upstream regulators, co-activators, co-repressors, and post-transcriptional regulators that influence miRNA synthesis, stability, and functionality. G protein-coupled receptors may promote and inhibit miR transcription, as other transcription factors such as p53 and MYC are implicated in the transcriptional activation or repression of apoptosis-related pathways and gene networks [88]. An interesting research showed that other epigenetic changes, including DNA methyltransferases and histone-modifying enzymes, might influence the promoter of miRNA genes, therefore either silencing or activating specific miRs [89],[90]. Moreover, RNA-binding proteins, including Argonaute (AGO) proteins, are essential to form the RNA-induced silencing complex, which is necessary for target recognition with miR and facilitates either mRNA destruction or translational repression [91]. Furthermore, crosstalking ceRNAs, such as lncRNA and circRNA, function as miRNA sponges, hence modulating the availability of miR to its target mRNAs, which are reviewed in the literature [92]. The several levels of control illustrate the intricacy of miRNA-mediated gene silencing and emphasize the need to explore upstream regulators and co-factors to effectively harness miRNAs as therapeutic targets in breast cancer.
Apoptosis is essential for the development and upkeep of multicellular creatures, as it eliminates cells that are damaged, aged, or affected by autoimmune conditions [93]. The last stages of apoptosis entail initiating cell death proteases, which trigger a series of protein breakdown processes, including effector caspases (Figure 3). Cancer cells can avoid apoptosis, which allows them to survive in challenging conditions, unlike healthy cells. They accomplish this through several means, such as modifying the balance between apoptotic molecules. Significantly, the balance is linked to the proliferation of tumors and their capacity to withstand the effects of drugs such as cisplatin (DDP) and paclitaxel [94].
Cancer cells can adjust the equilibrium between pro-apoptotic and antiapoptotic factors to avoid undergoing apoptosis [95]. Research has demonstrated that the BCL-2 protein is frequently expressed at higher levels in the cytoplasm of fully developed BC cells. At the same time, its expression is lower in less specialized cancer cells [96]. These findings indicate that the modulation of BCL-2 is vital in controlling apoptosis and suppressing tumor growth, thus impacting the destiny of BC cells [97]. Undifferentiated BC cells are more susceptible to apoptosis compared to well-differentiated cells [98].
The BCL-2 and BCL-XL families have attracted interest as targets for therapy because of their essential functions in controlling cell death. Scientists have investigated different approaches to regulate apoptosis, such as retroviral systems, alterations to the localization machinery, antibodies that impede action, RNA interference (RNAi), and miRNAs [99]. Nevertheless, BH3-mimetic drugs have been demonstrated to be the most efficacious strategy to specifically target the BCL-2 family. Comprehending the molecules implicated in apoptosis is essential advance novel or enhanced chemotherapies [100].
miRs' swift post-transcriptional impacts and capacity to control numerous target genes, including transcription factors, may present superior therapeutic possibilities to address neurological illnesses compared to medications that focus on a solitary gene. Nevertheless, the specific cells and the physiological or pathological circumstances in which the miR family affects the BCL-2 family proteins still need to be better understood. Although pretreatment with miRs has shown efficacy in animal models' cerebral ischemia, conducting tests on these treatments is still essential as a vital part of developing a therapy for neurological illnesses. Several miRs are being tested in clinical trials for liver illnesses, thereby indicating that creating and administering miR therapeutics for new disease situations or targets will be possible. However, delivering miR treatments to the central nervous system (CNS) presents substantial difficulties and remains a crucial barrier to therapeutic use. However, specific challenges about miR-based therapeutics remain pertinent and must be addressed to enable their practical implementation in clinical settings: miRs exhibit instability in bodily fluids due to degradation by nucleases; thus, developing ways to enhance their stability is necessary. Several chemical changes, including 2′-O-methylation, phosphorothioate backbones, and locked nucleic acids (LNAs), have been shown to improve the stability of miR while preserving its biological activity. Thus, encapsulation in lipid nanoparticles, polymeric carriers, or exosomes offers enhanced protections against degradation while facilitating targeted administration [101],[102]. The ultimate problem pertains to off-target effects that arise from the ability of miRs to bind to several mRs since they just use a segment of their sequence for this interaction, potentially leading to gene silence in unintended targets. Recent advancements in computational target prediction and experimental validation enhance the sequence selectivity and minimize off-target effects. Moreover, alterations in the seed area of miR mimics and antagomiRs have improved the targeting efficacy [103]. A further issue in delivering miRs to the CNS is that the blood-brain barrier impedes this distribution. Recent techniques using nanoparticles, liposomes, and Adeno-Associated Viruses have shown enhanced CNS uptake by receptor-mediated transcytosis and direct stereotaxic injection [104]. Despite the positive outcomes demonstrated in preclinical investigations, additional advancements in the delivery methods, dose regulation, and safety concerns remain important. Addressing these difficulties will be essential to realize the full potential of miR therapies in neurological and other illnesses.
Notwithstanding these advancements, several challenges and inquiries remain unresolved, thus continuing to stimulate researchers' interest. A comprehensive knowledge of the context-dependent regulation of miRs and the influence of various cellular and physiological conditions on their targets, including the BCL-2 family, is essential. Comprehending miR's biology and regulation may reveal novel gene expression regulatory mechanisms and potential therapeutic targets. Nonetheless, the precise delivery mechanisms for miR-based therapeutics remain in the developing phase, particularly for targeting the CNS due to obstacles such as the blood-brain barrier. Cancer-targeted nanoparticles, exosome-based drug delivery systems, and ligand-conjugated carriers have promise; nevertheless, more research is necessary to improve the targeting efficacy and minimize adverse effects. Comprehensive clinical trials are required to evaluate miR therapeutics' long-term safety profiles and potential immune responses. Concurrently, creating novel miR bioinformatics resources to predict and experimentally validate the targets of these therapeutics is essential to reduce the off-target effects. From a translational perspective, using miRs with conventional chemotherapy, radiation, and immunotherapy may provide synergistic benefits and reduce resistance in certain aggressive malignancies. Moreover, discovering miR expression patterns in patients may facilitate the establishment of biomarkers for diagnosis, prognosis, and therapy response monitoring, thus representing a crucial advancement toward personalized treatment strategies. The observed gaps provide avenues for future research, thus expanding the therapeutic uses of miRs and presenting novel strategies for conventional challenges in treating cancer and neurological illnesses. This review emphasizes the innovative role of miRNA regulation and its intricate involvement in apoptosis, thus offering guidance for future research to leverage these insights for therapeutic advancements.
The primary objective of BC research has been to comprehend the etiology and mechanisms underlying the neoplastic alterations that impact the epithelial tissue of the mammary gland. While post-transcriptional mechanisms are important, recent research has demonstrated that miRs are crucial in regulating BC. Lately, the significance of BCL-2 in BC has been acknowledged, as miRs can either suppress or stimulate cancer development. Studies suggest that cancer cells display varying levels of miRs, which can either increase or decrease the expression of the BCL-2 protein family. This control has a significant effect on the prediction of the course of a disease and assists in ensuring consistent levels of gene expression in various types of cancers. Scientists have discovered that monitoring the level of BCL-2 expression can be utilized to monitor a patients' progress and identify the most optimal therapy approaches. While numerous miRs have been found that can selectively target and influence specific genes differently, further investigation is required to establish definitive findings and comprehensively comprehend the function of miRs in controlling the BCL-2 family proteins in treating BC.
TA and KMS designed the study; KM performed data collection and literature analysis; SV prepared figures and tables; TA and KMS prepared initial draft; KMS contributed to review and editing. All authors approved the final version of the manuscript.
The authors declare they have not used Artificial Intelligence (AI) tools in the creation of this article.
[1] |
Wilkinson L, Gathani T (2022) Understanding breast cancer as a global health concern. Br J Radiol 95: 20211033. https://doi.org/10.1259/bjr.20211033 ![]() |
[2] |
Bland KA, Mustafa R, McTaggart-Cowan H (2023) Patient preferences in metastatic breast cancer care: A scoping review. Cancers 15: 4331. https://doi.org/10.3390/cancers15174331 ![]() |
[3] |
Bhushan A, Gonsalves A, Menon JU (2021) Current state of breast cancer diagnosis, treatment, and theranostics. Pharmaceutics 13: 723. https://doi.org/10.3390/pharmaceutics13050723 ![]() |
[4] |
Pankotai-Bodó G, Oláh-Németh O, Sükösd F, et al. (2024) Routine molecular applications and recent advances in breast cancer diagnostics. J Biotechnol 380: 20-28. https://doi.org/10.1016/j.jbiotec.2023.12.005 ![]() |
[5] |
Amethiya Y, Pipariya P, Patel S, et al. (2022) Comparative analysis of breast cancer detection using machine learning and biosensors. Intell Med 2: 69-81. https://doi.org/10.1016/j.imed.2021.08.004 ![]() |
[6] |
Kandhavelu J, Subramanian K, Naidoo V, et al. (2024) A novel EGFR inhibitor, HNPMI, regulates apoptosis and oncogenesis by modulating BCL-2/BAX and p53 in colon cancer. Br J Pharmacol 181: 107-124. https://doi.org/10.1111/bph.16141 ![]() |
[7] |
Sundaram KKM, Bupesh G, Saravanan KM (2022) Instrumentals behind embryo and cancer: A platform for prospective future in cancer research. AIMS Mol Sci 9: 25-45. https://doi.org/10.3934/molsci.2022002 ![]() |
[8] |
Feng Y, Spezia M, Huang S, et al. (2018) Breast cancer development and progression: Risk factors, cancer stem cells, signaling pathways, genomics, and molecular pathogenesis. Genes Dis 5: 77-106. https://doi.org/10.1016/j.gendis.2018.05.001 ![]() |
[9] |
Qian S, Wei Z, Yang W, et al. (2022) The role of BCL-2 family proteins in regulating apoptosis and cancer therapy. Front Oncol 12: 985363. https://doi.org/10.3389/fonc.2022.985363 ![]() |
[10] |
Debela DT, Muzazu SG, Heraro KD, et al. (2021) New approaches and procedures for cancer treatment: Current perspectives. SAGE Open Med 9: 20503121211034366. https://doi.org/10.1177/20503121211034366 ![]() |
[11] |
Carlson RW, Anderson BO, Chopra R, et al. (2003) Treatment of breast cancer in countries with limited resources. Breast J 9: S67-S74. https://doi.org/10.1046/j.1524-4741.9.s2.6.x ![]() |
[12] |
Bamodu OA, Chung CC, Pisanic TR, et al. (2024) The intricate interplay between cancer stem cells and cell-of-origin of cancer: Implications for therapeutic strategies. Front Oncol 14: 1404628. https://doi.org/10.3389/fonc.2024.1404628 ![]() |
[13] |
Bhat GR, Sethi I, Sadida HQ, et al. (2024) Cancer cell plasticity: From cellular, molecular, and genetic mechanisms to tumor heterogeneity and drug resistance. Cancer Metastasis Rev 43: 197-228. https://doi.org/10.1007/s10555-024-10172-z ![]() |
[14] |
Galluzzi L, Vitale I, Aaronson SA, et al. (2018) Molecular mechanisms of cell death: Recommendations of the Nomenclature Committee on Cell Death 2018. Cell Death Differ 25: 486-541. https://doi.org/10.1038/s41418-017-0012-4 ![]() |
[15] |
Warren CFA, Wong-Brown MW, Bowden NA (2019) BCL-2 family isoforms in apoptosis and cancer. Cell Death Dis 10: 177. https://doi.org/10.1038/s41419-019-1407-6 ![]() |
[16] |
Rabkin CS, Hirt C, Janz S, et al. (2008) t(14;18) Translocations and risk of follicular lymphoma. JNCI Monogr 2008: 48-51. https://doi.org/10.1093/jncimonographs/lgn002 ![]() |
[17] |
Roulland S, Lebailly P, Lecluse Y, et al. (2004) Characterization of the t(14;18) BCL2-IGH translocation in farmers occupationally exposed to pesticides. Cancer Res 64: 2264-2269. https://doi.org/10.1158/0008-5472.can-03-3604 ![]() |
[18] |
Singh R, Letai A, Sarosiek K (2019) Regulation of apoptosis in health and disease: The balancing act of BCL-2 family proteins. Nat Rev Mol Cell Biol 20: 175-193. https://doi.org/10.1038/s41580-018-0089-8 ![]() |
[19] |
Banjara S, Suraweera CD, Hinds MG, et al. (2020) The BCL-2 family: Ancient origins, conserved structures, and divergent mechanisms. Biomolecules 10: 128. https://doi.org/10.3390/biom10010128 ![]() |
[20] |
Zografos E, Zagouri F, Kalapanida D, et al. (2019) Prognostic role of microRNAs in breast cancer: A systematic review. Oncotarget 10: 7156-7178. https://doi.org/10.18632/oncotarget.27327 ![]() |
[21] |
Muñoz JP, Pérez-Moreno P, Pérez Y, et al. (2023) The role of microRNAs in breast cancer and the challenges of their clinical application. Diagnostics 13: 3072. https://doi.org/10.3390/diagnostics13193072 ![]() |
[22] |
Sharma PC, Gupta A (2020) MicroRNAs: Potential biomarkers for diagnosis and prognosis of different cancers. Transl Cancer Res 9: 5798-5818. https://doi.org/10.21037/tcr-20-1294 ![]() |
[23] |
Ying SY, Chang DC, Lin SL (2008) The microRNA (miRNA): Overview of the RNA genes that modulate gene function. Mol Biotechnol 38: 257-268. https://doi.org/10.1007/s12033-007-9013-8 ![]() |
[24] |
Pekarek L, Torres-Carranza D, Fraile-Martinez O, et al. (2023) An overview of the role of microRNAs on carcinogenesis: A focus on cell cycle, angiogenesis and metastasis. Int J Mol Sci 24: 7268. https://doi.org/10.3390/ijms24087268 ![]() |
[25] |
Sharifi Z, Talkhabi M, Taleahmad S (2022) Identification of potential microRNA diagnostic panels and uncovering regulatory mechanisms in breast cancer pathogenesis. Sci Rep 12: 20135. https://doi.org/10.1038/s41598-022-24347-7 ![]() |
[26] |
Abdul Manap AS, Wisham AA, Wong FW, et al. (2024) Mapping the function of microRNAs as a critical regulator of tumor-immune cell communication in breast cancer and potential treatment strategies. Front Cell Dev Biol 12: 1390704. https://doi.org/10.3389/fcell.2024.1390704 ![]() |
[27] |
Kaloni D, Diepstraten ST, Strasser A, et al. (2023) BCL-2 protein family: Attractive targets for cancer therapy. Apoptosis 28: 20-38. https://doi.org/10.1007/s10495-022-01780-7 ![]() |
[28] |
O'Day E, Lal A (2010) MicroRNAs and their target gene networks in breast cancer. Breast Cancer Res 12: 201. https://doi.org/10.1186/bcr2484 ![]() |
[29] |
Saddam M, Paul SK, Habib MA, et al. (2024) Emerging biomarkers and potential therapeutics of the BCL-2 protein family: The apoptotic and antiapoptotic context. Egypt J Med Hum Genet 25: 12. https://doi.org/10.1186/s43042-024-00485-7 ![]() |
[30] |
Wang H, Guo M, Wei H, et al. (2023) Targeting p53 pathways: Mechanisms, structures, and advances in therapy. Sig Transduct Target Ther 8: 92. https://doi.org/10.1038/s41392-023-01347-1 ![]() |
[31] |
Elmore S (2007) Apoptosis: A review of programmed cell death. Toxicol Pathol 35: 495-516. https://doi.org/10.1080/01926230701320337 ![]() |
[32] |
Jan R, Chaudhry G (2019) Understanding apoptosis and apoptotic pathways targeted cancer therapeutics. Adv Pharm Bull 9: 205-218. https://doi.org/10.15171/apb.2019.024 ![]() |
[33] |
Kale J, Osterlund EJ, Andrews DW (2018) BCL-2 family proteins: Changing partners in the dance towards death. Cell Death Differ 25: 65-80. https://doi.org/10.1038/cdd.2017.186 ![]() |
[34] |
Letai A, Bassik MC, Walensky LD, et al. (2002) Distinct BH3 domains either sensitize or activate mitochondrial apoptosis, serving as prototype cancer therapeutics. Cancer Cell 2: 183-192. https://doi.org/10.1016/S1535-6108(02)00127-7 ![]() |
[35] |
Lomonosova E, Chinnadurai G (2008) BH3-only proteins in apoptosis and beyond: An overview. Oncogene 27: S2-S19. https://doi.org/10.1038/onc.2009.39 ![]() |
[36] |
Lalier L, Vallette F, Manon S (2022) BCL-2 family members and the mitochondrial import machineries: The roads to death. Biomolecules 12: 162. https://doi.org/10.3390/biom12020162 ![]() |
[37] |
Chen D, Frezza M, Schmitt S, et al. (2011) Bortezomib as the first proteasome inhibitor anticancer drug: Current status and future perspectives. Curr Cancer Drug Targets 11: 239-253. https://doi.org/10.2174/156800911794519752 ![]() |
[38] |
Harris MH, Thompson CB (2000) The role of the BCL-2 family in the regulation of outer mitochondrial membrane permeability. Cell Death Differ 7: 1182-1191. https://doi.org/10.1038/sj.cdd.4400781 ![]() |
[39] |
Jang DM, Oh EK, Hahn H, et al. (2022) Structural insights into apoptotic regulation of human Bfk as a novel BCL-2 family member. Comput Struct Biotechnol J 20: 745-756. https://doi.org/10.1016/j.csbj.2022.01.023 ![]() |
[40] |
Kelekar A, Thompson CB (1998) BCL-2-family proteins: The role of the BH3 domain in apoptosis. Trends Cell Biol 8: 324-330. https://doi.org/10.1016/s0962-8924(98)01321-x ![]() |
[41] |
Dadsena S, Jenner A, García-Sáez AJ (2021) Mitochondrial outer membrane permeabilization at the single molecule level. Cell Mol Life Sci 78: 3777-3790. https://doi.org/10.1007/s00018-021-03771-4 ![]() |
[42] |
Bender T, Martinou JC (2013) Where killers meet—permeabilization of the outer mitochondrial membrane during apoptosis. Cold Spring Harb Perspect Biol 5: a011106. https://doi.org/10.1101/cshperspect.a011106 ![]() |
[43] |
Czabotar PE, Garcia-Saez AJ (2023) Mechanisms of BCL-2 family proteins in mitochondrial apoptosis. Nat Rev Mol Cell Biol 24: 732-748. https://doi.org/10.1038/s41580-023-00629-4 ![]() |
[44] |
Peña-Blanco A, García-Sáez AJ (2018) Bax, Bak and beyond—mitochondrial performance in apoptosis. FEBS J 285: 416-431. https://doi.org/10.1111/febs.14186 ![]() |
[45] |
MacFarlane LA, Murphy PR (2010) MicroRNA: Biogenesis, function and role in cancer. Curr Genomics 11: 537-561. https://doi.org/10.2174/138920210793175895 ![]() |
[46] |
Starega-Roslan J, Koscianska E, Kozlowski P, et al. (2011) The role of the precursor structure in the biogenesis of microRNA. Cell Mol Life Sci 68: 2859-2871. https://doi.org/10.1007/s00018-011-0726-2 ![]() |
[47] |
O'Brien J, Hayder H, Zayed Y, et al. (2018) Overview of microRNA biogenesis, mechanisms of actions, and circulation. Front Endocrinol 9: 402. https://doi.org/10.3389/fendo.2018.00402 ![]() |
[48] |
Haas G, Cetin S, Messmer M, et al. (2016) Identification of factors involved in target RNA-directed microRNA degradation. Nucleic Acids Res 44: 2873-2887. https://doi.org/10.1093/nar/gkw040 ![]() |
[49] | Bujak M, Ratkaj I, Baus M, et al. (2011) Small interfering RNAs: Heralding a new era in gene therapy. Gene therapy—developments and future perspectives.IntechOpen. https://doi.org/10.5772/20646 |
[50] |
Gu S, Jin L, Zhang F, et al. (2009) Biological basis for restriction of microRNA targets to the 3′ untranslated region in mammalian mRNAs. Nat Struct Mol Biol 16: 144-150. https://doi.org/10.1038/nsmb.1552 ![]() |
[51] |
Menon A, Abd-Aziz N, Khalid K, et al. (2022) miRNA: A promising therapeutic target in cancer. Int J Mol Sci 23: 11502. https://doi.org/10.3390/ijms231911502 ![]() |
[52] |
Peng Y, Croce CM (2016) The role of microRNAs in human cancer. Signal Transduct Target Ther 1: 15004. https://doi.org/10.1038/sigtrans.2015.4 ![]() |
[53] |
Otmani K, Lewalle P (2021) Tumor suppressor miRNA in cancer cells and the tumor microenvironment: Mechanism of deregulation and clinical implications. Front Oncol 11: 708765. https://doi.org/10.3389/fonc.2021.708765 ![]() |
[54] |
Spadaccino F, Gigante M, Netti GS, et al. (2021) The ambivalent role of miRNAs in carcinogenesis: Involvement in renal cell carcinoma and their clinical applications. Pharmaceuticals 14: 322. https://doi.org/10.3390/ph14040322 ![]() |
[55] |
Shah MY, Ferrajoli A, Sood AK, et al. (2016) microRNA therapeutics in cancer—An emerging concept. EBioMedicine 12: 34-42. https://doi.org/10.1016/j.ebiom.2016.09.017 ![]() |
[56] |
Bayir H, Kagan VE (2008) Bench-to-bedside review: Mitochondrial injury, oxidative stress and apoptosis—there is nothing more practical than a good theory. Crit Care 12: 206. https://doi.org/10.1186/cc6779 ![]() |
[57] |
Garrido C, Galluzzi L, Brunet M, et al. (2006) Mechanisms of cytochrome c release from mitochondria. Cell Death Differ 13: 1423-1433. https://doi.org/10.1038/sj.cdd.4401950 ![]() |
[58] |
Adams JM, Cory S (2018) The BCL-2 arbiters of apoptosis and their growing role as cancer targets. Cell Death Differ 25: 27-36. https://doi.org/10.1038/cdd.2017.161 ![]() |
[59] |
Garrido-Cano I, Pattanayak B, Adam-Artigues A, et al. (2022) MicroRNAs as a clue to overcome breast cancer treatment resistance. Cancer Metastasis Rev 41: 77-105. https://doi.org/10.1007/s10555-021-09992-0 ![]() |
[60] |
Chakrabortty A, Patton DJ, Smith BF, et al. (2023) miRNAs: Potential as biomarkers and therapeutic targets for cancer. Genes 14: 1375. https://doi.org/10.3390/genes14071375 ![]() |
[61] |
Hong T, Ding J, Li W (2019) mir-7 reverses breast cancer resistance to chemotherapy by targeting MRP1 and BCL2. Onco Targets Ther 12: 11097-11105. https://doi.org/10.2147/OTT.S213780 ![]() |
[62] |
Cittelly DM, Das PM, Salvo VA, et al. (2010) Oncogenic HER2Δ16 suppresses miR-15a/16 and deregulates BCL-2 to promote endocrine resistance of breast tumors. Carcinogenesis 31: 2049-2057. https://doi.org/10.1093/carcin/bgq192 ![]() |
[63] |
Tao L, Wu YQ, Zhang SP (2019) MiR-21-5p enhances the progression and paclitaxel resistance in drug-resistant breast cancer cell lines by targeting PDCD4. Neoplasma 66: 746-755. https://doi.org/10.4149/neo_2018_181207N930 ![]() |
[64] |
Manvati S, Mangalhara KC, Kalaiarasan P, et al. (2015) miR-24-2 regulates genes in survival pathway and demonstrates potential in reducing cellular viability in combination with docetaxel. Gene 567: 217-224. https://doi.org/10.1016/j.gene.2015.05.003 ![]() |
[65] |
Han X, Li Q, Liu C, et al. (2019) Overexpression miR-24-3p repressed Bim expression to confer tamoxifen resistance in breast cancer. J Cell Biochem 120: 12966-12976. https://doi.org/10.1002/jcb.28568 ![]() |
[66] |
Körner C, Keklikoglou I, Bender C, et al. (2013) MicroRNA-31 sensitizes human breast cells to apoptosis by direct targeting of protein Kinase C ϵ (PKCϵ). J Biol Chem 288: 8750-8761. ![]() |
[67] |
Kastl L, Brown I, Schofield AC (2012) miRNA-34a is associated with docetaxel resistance in human breast cancer cells. Breast Cancer Res Treat 131: 445-454. https://doi.org/10.1007/s10549-011-1424-3 ![]() |
[68] |
Bao C, Chen J, Chen D, et al. (2020) MiR-93 suppresses tumorigenesis and enhances chemosensitivity of breast cancer via dual targeting E2F1 and CCND1. Cell Death Dis 11: 618. https://doi.org/10.1038/s41419-020-02855-6 ![]() |
[69] |
You F, Luan H, Sun D, et al. (2018) miRNA-106a promotes breast cancer cell proliferation, clonogenicity, migration, and invasion through inhibiting apoptosis and chemosensitivity. DNA Cell Biol 38: 198-207. https://doi.org/10.1089/dna.2018.4282 ![]() |
[70] |
Zhang W, Jiang H, Chen Y, et al. (2019) Resveratrol chemosensitizes adriamycin-resistant breast cancer cells by modulating miR-122-5p. J Cell Biochem 120: 16283-16292. https://doi.org/10.1002/jcb.28910 ![]() |
[71] |
Xie X, Hu Y, Xu L, et al. (2015) The role of miR-125b-mitochondria-caspase-3 pathway in doxorubicin resistance and therapy in human breast cancer. Tumor Biol 36: 7185-7194. https://doi.org/10.1007/s13277-015-3438-7 ![]() |
[72] |
Zhou M, Liu Z, Zhao Y, et al. (2010) MicroRNA-125b confers the resistance of breast cancer cells to paclitaxel through suppression of pro-apoptotic BCL-2 antagonist killer 1 (Bak1) expression. J Biol Chem 285: 21496-21507. https://doi.org/10.1074/jbc.M109.083337 ![]() |
[73] |
O'Brien K, Lowry MC, Corcoran C, et al. (2015) miR-134 in extracellular vesicles reduces triple-negative breast cancer aggression and increases drug sensitivity. Oncotarget 6: 32774-32789. https://doi.org/10.18632/oncotarget.5192 ![]() |
[74] |
Xiang F, Fan Y, Ni Z, et al. (2019) Ursolic acid reverses the chemoresistance of breast cancer cells to paclitaxel by targeting miRNA-149-5p/MyD88. Front Oncol 9: 00501. https://doi.org/10.3389/fonc.2019.00501 ![]() |
[75] |
Zheng Y, Lv X, Wang X, et al. (2016) miR-181b promotes chemoresistance in breast cancer by regulating Bim expression. Oncol Rep 35: 683-690. https://doi.org/10.3892/or.2015.4417 ![]() |
[76] |
Zhang Y, He Y, Lu LL, et al. (2019) miRNA-192-5p impacts the sensitivity of breast cancer cells to doxorubicin via targeting peptidylprolyl isomerase A. Kaohsiung J Med Sci 35: 17-23. https://doi.org/10.1002/kjm2.12004 ![]() |
[77] |
Yang G, Wu D, Zhu J, et al. (2013) Upregulation of miR-195 increases the sensitivity of breast cancer cells to Adriamycin treatment through inhibition of Raf-1. Oncol Rep 30: 877-889. https://doi.org/10.3892/or.2013.2532 ![]() |
[78] |
Aakko S, Straume AH, Birkeland EE, et al. (2019) MYC-induced miR-203b-3p and miR-203a-3p control BCL-xL expression and paclitaxel sensitivity in tumor cells. Transl Oncol 12: 170-179. https://doi.org/10.1016/j.tranon.2018.10.001 ![]() |
[79] |
Ye Z, Hao R, Cai Y, et al. (2016) Knockdown of miR-221 promotes the cisplatin-inducing apoptosis by targeting the BIM-Bax/Bak axis in breast cancer. Tumor Biol 37: 4509-4515. https://doi.org/10.1007/s13277-015-4267-4 ![]() |
[80] |
Dai H, Xu L, Qian Q, et al. (2019) MicroRNA-222 promotes drug resistance to doxorubicin in breast cancer via regulation of miR-222/bim pathway. Biosci Rep 39: BSR20190650. https://doi.org/10.1042/BSR20190650 ![]() |
[81] |
Gu X, Li JY, Guo J, et al. (2015) Influence of MiR-451 on drug resistances of paclitaxel-resistant breast cancer cell line. Med Sci Monit 21: 3291-3297. https://doi.org/10.12659/msm.894475 ![]() |
[82] |
Chen X, Wang YW, Xing AY, et al. (2016) Suppression of SPIN1-mediated PI3K–Akt pathway by miR-489 increases chemosensitivity in breast cancer. J Pathol 239: 459-472. https://doi.org/10.1002/path.4743 ![]() |
[83] | Jiang H, Cheng L, Hu P, et al. (2018) MicroRNA‑663b mediates TAM resistance in breast cancer by modulating TP73 expression. Mol Med Rep 18: 1120-1126. https://doi.org/10.3892/mmr.2018.9064 |
[84] |
Ito Y, Inoue A, Seers T, et al. (2017) Identification of targets of tumor suppressor microRNA-34a using a reporter library system. Proc Natl Acad Sci 114: 3927-3932. https://doi.org/10.1073/pnas.1620019114 ![]() |
[85] |
Cimmino A, Calin GA, Fabbri M, et al. (2005) miR-15 and miR-16 induce apoptosis by targeting BCL2. Proc Natl Acad Sci U S A 102: 13944-13949. https://doi.org/10.1073/pnas.0506654102 ![]() |
[86] |
Sims EK, Lakhter AJ, Anderson-Baucum E, et al. (2017) MicroRNA 21 targets BCL2 mRNA to increase apoptosis in rat and human beta cells. Diabetologia 60: 1057-1065. https://doi.org/10.1007/s00125-017-4237-z ![]() |
[87] |
Garrido-Palacios A, Rojas Carvajal AM, Núñez-Negrillo AM, et al. (2023) MicroRNA dysregulation in early breast cancer diagnosis: A systematic review and meta-analysis. Int J Mol Sci 24: 8270. https://doi.org/10.3390/ijms24098270 ![]() |
[88] |
Capaccia C, Diverio S, Zampini D, et al. (2022) The complex interaction between P53 and miRNAs joins new awareness in physiological stress responses. Cells 11: 1631. https://doi.org/10.3390/cells11101631 ![]() |
[89] |
Arif KMT, Elliott EK, Haupt LM, et al. (2020) Regulatory mechanisms of epigenetic miRNA relationships in human cancer and potential as therapeutic targets. Cancers 12: 2922. https://doi.org/10.3390/cancers12102922 ![]() |
[90] |
Calin GA, Dumitru CD, Shimizu M, et al. (2002) Frequent deletions and down-regulation of micro-RNA genes miR15 and miR16 at 13q14 in chronic lymphocytic leukemia. Proc Natl Acad Sci U S A 99: 15524-15529. https://doi.org/10.1073/pnas.242606799 ![]() |
[91] |
Peters L, Meister G (2007) Argonaute proteins: Mediators of RNA silencing. Mol Cell 26: 611-623. https://doi.org/10.1016/j.molcel.2007.05.001 ![]() |
[92] |
Ma B, Wang S, Wu W, et al. (2023) Mechanisms of circRNA/lncRNA-miRNA interactions and applications in disease and drug research. Biomed Pharmacother 162: 114672. https://doi.org/10.1016/j.biopha.2023.114672 ![]() |
[93] |
Fadeel B, Orrenius S (2005) Apoptosis: A basic biological phenomenon with wide-ranging implications in human disease. J Intern Med 258: 479-517. https://doi.org/10.1111/j.1365-2796.2005.01570.x ![]() |
[94] |
Si W, Shen J, Zheng H, et al. (2019) The role and mechanisms of action of microRNAs in cancer drug resistance. Clin Epigenet 11: 25. https://doi.org/10.1186/s13148-018-0587-8 ![]() |
[95] |
Mohammad RM, Muqbil I, Lowe L, et al. (2015) Broad targeting of resistance to apoptosis in cancer. Semin Cancer Biol 35: S78-S103. https://doi.org/10.1016/j.semcancer.2015.03.001 ![]() |
[96] | Callens M, Kraskovskaya N, Derevtsova K, et al. (2021) The role of BCL-2 proteins in modulating neuronal Ca2+ signaling in health and in Alzheimer's disease. BBA-Mol Cell Res 1868: 118997. https://doi.org/10.1016/j.bbamcr.2021.118997 |
[97] |
D'Aguanno S, Brignone M, Scalera S, et al. (2024) BCL-2 dependent modulation of Hippo pathway in cancer cells. Cell Commun Signal 22: 277. https://doi.org/10.1186/s12964-024-01647-1 ![]() |
[98] | Abaza A, Vasavada AM, Sadhu A, et al. (2022) A systematic review of apoptosis in correlation with cancer: Should apoptosis be the ultimate target for cancer treatment?. Cureus 14: e28496. https://doi.org/10.7759/cureus.28496 |
[99] | Huska J, Lamb HM, Hardwick JM (2019) Overview of BCL-2 family proteins and therapeutic potentials. BCL-2 family proteins. Methods in molecular biology. New York: Humana Press 1-21. https://doi.org/10.1007/978-1-4939-8861-7_1 |
[100] |
Yap JL, Chen L, Lanning ME, et al. (2017) Expanding the cancer arsenal with targeted therapies: Disarmament of the antiapoptotic BCL-2 proteins by small molecules. J Med Chem 60: 821-838. https://doi.org/10.1021/acs.jmedchem.5b01888 ![]() |
[101] |
Tassone P, Di Martino MT, Arbitrio M, et al. (2023) Safety and activity of the first-in-class locked nucleic acid (LNA) miR-221 selective inhibitor in refractory advanced cancer patients: a first-in-human, phase 1, open-label, dose-escalation study. J Hematol Oncol 16: 68. https://doi.org/10.1186/s13045-023-01468-8 ![]() |
[102] |
Qassem S, Breier D, Naidu GS, et al. (2024) Unlocking the therapeutic potential of locked nucleic acids through lipid nanoparticle delivery. Mol Ther Nucl Acids 35: 102224. https://doi.org/10.1016/j.omtn.2024.102224 ![]() |
[103] |
Riolo G, Cantara S, Marzocchi C, et al. (2020) miRNA targets: From prediction tools to experimental validation. Methods Protoc 4: 1. https://doi.org/10.3390/mps4010001 ![]() |
[104] |
Hersh AM, Alomari S, Tyler BM (2022) Crossing the blood-brain barrier: Advances in nanoparticle technology for drug delivery in neuro-oncology. Int J Mol Sci 23: 4153. https://doi.org/10.3390/ijms23084153 ![]() |
Target gene | miR | Regulation | Reference |
BCL-2 | miR-7 | Inhibits target BCL-2 gene directly | [61] |
BCL-2 | miR-15a/16 | Inhibits target BCL-2 gene directly | [62] |
BCL-2 | miR-21 | Activates target BCL-2 gene directly | [63] |
BCL-2 | miR-24–2 | Inhibits target BCL-2gene directly | [64] |
BIM | miR-24-3p | Inhibits target BIM gene directly | [65] |
BCL-2 | miR-31 | Inhibits target BCL-2 gene directly | [66] |
BCL-2 | miR-34a | Inhibits target BCL-2 gene directly | [67] |
BCL-2 BAX |
miR-93 | Inhibits target BCL-2 gene directly Inhibits target BAX gene directly |
[68] |
BCL-2 | miR-106 | Activates target BCL-2 gene directly | [69] |
BCL-2 | miR-122-5p | Inhibits target BCL-2gene directly | [70] |
BCL-2 BAK1 |
miR-125b | Inhibits target BCL-2 gene directly Inhibits target BAK1 gene directly |
[71],[72] |
BCL-2 | miR-134 | Inhibits target BCL-2 gene directly | [73] |
BAX | miR-149-5p | Inhibits target BAX gene directly | [74] |
BIM | miR-181b | Inhibits target BIM gene directly | [75] |
BAD | miR-192-5b | Inhibits target BAD gene directly Inhibits target BCL-2 gene indirectly |
[76] |
BCL-2 | miR-195 | Inhibits target BCL-2 gene directly | [77] |
BCL-XL | miR-203a-3p miR-203b-3p |
Inhibits target BCL-XL gene directly Inhibits target BCL-XL gene directly |
[78] |
BIM BAX BAK | miR-221 | Inhibits target BIM gene directly | [79] |
BIM | miR-222 | Inhibits target BIM gene directly | [80] |
BCL-2 | miR-451 | Inhibits target BCL-2 gene directly | [81] |
BCL-2 | miR-489 | Inhibits target BCL-2 gene directly | [82] |
BAX* | miR-663b | Activates target BCL-2 gene directly | [83] |
Target gene | miR | Regulation | Reference |
BCL-2 | miR-7 | Inhibits target BCL-2 gene directly | [61] |
BCL-2 | miR-15a/16 | Inhibits target BCL-2 gene directly | [62] |
BCL-2 | miR-21 | Activates target BCL-2 gene directly | [63] |
BCL-2 | miR-24–2 | Inhibits target BCL-2gene directly | [64] |
BIM | miR-24-3p | Inhibits target BIM gene directly | [65] |
BCL-2 | miR-31 | Inhibits target BCL-2 gene directly | [66] |
BCL-2 | miR-34a | Inhibits target BCL-2 gene directly | [67] |
BCL-2 BAX |
miR-93 | Inhibits target BCL-2 gene directly Inhibits target BAX gene directly |
[68] |
BCL-2 | miR-106 | Activates target BCL-2 gene directly | [69] |
BCL-2 | miR-122-5p | Inhibits target BCL-2gene directly | [70] |
BCL-2 BAK1 |
miR-125b | Inhibits target BCL-2 gene directly Inhibits target BAK1 gene directly |
[71],[72] |
BCL-2 | miR-134 | Inhibits target BCL-2 gene directly | [73] |
BAX | miR-149-5p | Inhibits target BAX gene directly | [74] |
BIM | miR-181b | Inhibits target BIM gene directly | [75] |
BAD | miR-192-5b | Inhibits target BAD gene directly Inhibits target BCL-2 gene indirectly |
[76] |
BCL-2 | miR-195 | Inhibits target BCL-2 gene directly | [77] |
BCL-XL | miR-203a-3p miR-203b-3p |
Inhibits target BCL-XL gene directly Inhibits target BCL-XL gene directly |
[78] |
BIM BAX BAK | miR-221 | Inhibits target BIM gene directly | [79] |
BIM | miR-222 | Inhibits target BIM gene directly | [80] |
BCL-2 | miR-451 | Inhibits target BCL-2 gene directly | [81] |
BCL-2 | miR-489 | Inhibits target BCL-2 gene directly | [82] |
BAX* | miR-663b | Activates target BCL-2 gene directly | [83] |