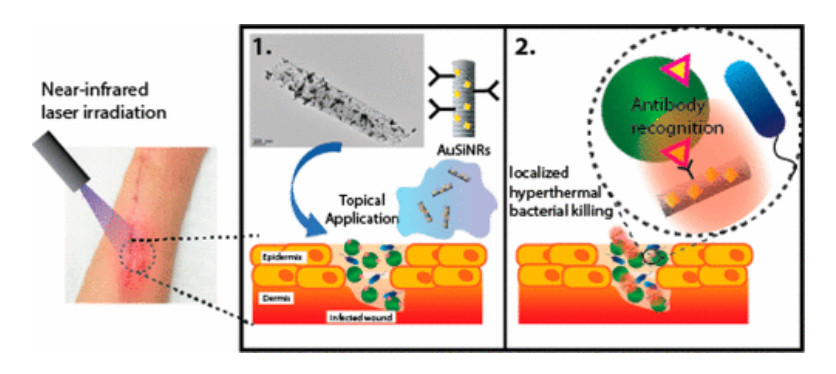
Surface engineering using nanopillars has been used for the fabrication of antimicrobial surfaces, cell culture environments that mimic the extracellular environment, and biosensors for the characterization of biomolecules. In this mini review, we summarize the roles of surface topography on various biochemical processes played by tunable nanopillars.
Citation: Sumaiya F. Begum, Hai-Feng Ji. Biochemistry tuned by nanopillars[J]. AIMS Materials Science, 2021, 8(5): 748-759. doi: 10.3934/matersci.2021045
[1] | Chandni Lotwala, Hai-Feng Ji . Electrochemistry on nanopillared electrodes. AIMS Materials Science, 2017, 4(2): 292-301. doi: 10.3934/matersci.2017.2.292 |
[2] | Hai-Feng Ji, Morasae Samadi, Hao Gu, Veronica Tomchak, Zhen Qiao . Fabrication and applications of self-assembled nanopillars. AIMS Materials Science, 2017, 4(4): 905-919. doi: 10.3934/matersci.2017.4.905 |
[3] | Lu Liao, Guo Li, Junjie Zhang . Experimental investigation of cutting parameters dependence in diamond turning of monocrystalline silicon. AIMS Materials Science, 2019, 6(5): 635-645. doi: 10.3934/matersci.2019.5.635 |
[4] | Yuan Guo, Xiong (Bill) Yu . Characterizing the surface charge of clay minerals with Atomic Force Microscope (AFM). AIMS Materials Science, 2017, 4(3): 582-593. doi: 10.3934/matersci.2017.3.582 |
[5] | Olayinka Oluwatosin Abegunde, Esther Titilayo Akinlabi, Oluseyi Philip Oladijo, Stephen Akinlabi, Albert Uchenna Ude . Overview of thin film deposition techniques. AIMS Materials Science, 2019, 6(2): 174-199. doi: 10.3934/matersci.2019.2.174 |
[6] | Jie Sun, Tzvetanka Boiadjieva-Scherzer, Hermann Kronberger, Kevin Staats, Johannes Holinka, Reinhard Windhager . Surface modification of Ti6Al4V alloy for implants by anodization and electrodeposition. AIMS Materials Science, 2019, 6(5): 713-729. doi: 10.3934/matersci.2019.5.713 |
[7] | Shuhan Jing, Adnan Younis, Dewei Chu, Sean Li . Resistive Switching Characteristics in Electrochemically Synthesized ZnO Films. AIMS Materials Science, 2015, 2(2): 28-36. doi: 10.3934/matersci.2015.2.28 |
[8] | Rasheed Atif, Fawad Inam . Influence of macro-topography on mechanical performance of 0.5 wt% nanoclay/multi-layer graphene-epoxy nanocomposites. AIMS Materials Science, 2016, 3(4): 1294-1308. doi: 10.3934/matersci.2016.4.1294 |
[9] | Hyeck Go, Eun-Mi Han, Moon Hee Kang, Yong Hyun Kim, Changhun Yun . The coated porous polyimide layers for optical scattering films. AIMS Materials Science, 2018, 5(6): 1102-1111. doi: 10.3934/matersci.2018.6.1102 |
[10] | Bandar Abdullah Aloyaydi, Subbarayan Sivasankaran, Hany Rizk Ammar . Influence of infill density on microstructure and flexural behavior of 3D printed PLA thermoplastic parts processed by fusion deposition modeling. AIMS Materials Science, 2019, 6(6): 1033-1048. doi: 10.3934/matersci.2019.6.1033 |
Surface engineering using nanopillars has been used for the fabrication of antimicrobial surfaces, cell culture environments that mimic the extracellular environment, and biosensors for the characterization of biomolecules. In this mini review, we summarize the roles of surface topography on various biochemical processes played by tunable nanopillars.
All solid materials contain a surface that interacts with other materials. The surface topography of these materials can be used to manipulate biochemical processes based on interactions with the biological environment. Nanostructured surfaces are currently used to control behaviors of cells and biomolecules with their environment at the cellular and macro-scale level [1]. Nanopillars, for example, are a major type of nanostructures on surfaces used to study the dynamics of biomolecules. Nanopillars are columnar structures arranged in a lattice array and consist of tunable dimensions, geometries, and chemical compositions that determine their overall mechanical properties [2]. Studies have varied the height, thickness, roughness, and chemical composition of the nanopillars to elucidate how the physical and chemical properties of the nanopillars affect interaction with biomolecules. For example, the mechanical properties of nanopillars have been applied in various biochemical studies from antimicrobial properties to controlled cell differentiation. In nature, many organisms use nanostructured surfaces to interact with biomolecules and other organisms. In this mini review, we aim to discuss how nanopillars have been used in biochemical applications such as antimicrobial, biosensing, biorecognition, and cell differentiation studies.
Antibiotic resistance of fast-evolving bacteria has become a growing concern. Antibiotic medications are a quick and low-cost method combating bacteria as antibiotics are readily available for use compared to other methods of treatment. However, the overuse of antibiotics often leads to bacterial resistance as the non-resistant strains are killed by the antibiotics, leaving behind the resistant colonies to further multiply and spread [3]. An alternative method would be to use other chemical treatments such as bacteriocins or antimicrobial peptides, but bacteria are capable of evolving resistance to the biochemical mechanism of these chemicals as well [4]. Furthermore, phage therapy, using bacteriophages to infect and lyse bacteria, was considered to hold promise for a better antibiotic method, but complications also arise from potential resistance and phage-mediated gene transfer [4]. The study of hyperthermally active nanomaterials is a novel field of research specialized in killing pathogenic organisms such as bacteria and fungi to neoplastic tissue in humans via induced hyperthermia mechanisms of the target. Hyperthermal activation involves heating various surfaces, such as maghemite nanomaterials, using radiofrequency wavelengths [5]. Other materials, such as magnetic iron oxide nanoparticles are also used for hyperthermal activation [6]. Although these nanomaterials show great potential in tumor ablation and destruction of pathogenic cells, they pose risks for host cells. Cells exposed to iron oxide nanomaterials have shown damage from oxidative stresses such as the formation of reactive oxygen species, disruption of mitochondrial function, and alteration of biomolecules and cell signaling pathways [7].Alternatively, some nanopillars, on the other hand, have high absorption coefficients in the near-IR (NIR) region, and thus, they are more promising antibacterial agents.
Hashim et al. applied laser irradiation on various types of engineered nanopillars to demonstrate how nanopillars can be used to control bacterial growth on surfaces [8]. They constructed silicon nanopillars (SiNP), silicon nanopillars decorated with gold nanoclusters (AuSiNP), and chemically modified AuSiNP bonded with an anti-S. aureus antibody (AuSiNP-Ab). An 808 nm laser was used to irradiate varying concentrations of the topically applied nanopillar samples in solutions, and S. aureus growth was monitored (Figure 1). Results showed that the laser irradiation on the SiNP at 1 mg/mL concentration had no effects on S. aureus growth since sufficient heating to stop the growth did not occur in the absence of gold nanoparticles (85–98% bacterial viability). Other concentrations of the SiNP (0.1 mg/mL and 0.5 mg/mL) also showed similar results to the control sample. On the contrary, both AuSiNP and AuSiNP-Ab resulted in less than 1% viability of S. aureus at 1.0 mg/mL concentration (Figure 2), demonstrating the enhanced antibacterial properties of the nanopillars (Figure 2). Additionally, the AuSiNP, SiNP, and AuSiNP-Ab samples showed no decrease in the host human foreskin fibroblast (HFF) viability. Scanning electron microscopy confirmed extensive damage to the bacterial cell wall and the cell shape from laser irradiation of AuSiNP, demonstrating the success of photothermally-activated nanopillars as antibacterial agents. This type of nanopillar technology shows promising antibacterial properties with little to no host cell damage where conventional treatments such as antibiotics fail against antibiotic-resistant pathogens.
For nanopillars on a surface, photothermal activation is not the only method needed to kill bacteria. Bandara et al. showed that engineered nanopillars can emulate natural dragonfly wing topography for antimicrobial properties. They determined that dragonfly wings consist of two types of protruding nanopillars with varying heights, and this topography confers antibacterial properties as host defense to the dragonfly [9]. This is important because engineered nanotextured surface models typically use nanopillars of a uniform height, but the dragonfly model displays antibacterial activity using nanopillars of varying heights. The effectiveness of the nanopillar topography was illustrated using the E. coli model. E. coli secrete extracellular polymeric adhesions (EPS), which is a sticky substance that adheres the bacterium to the wing surface of the host. However, this adhesion force is counterproductive to the bacteria as any movement initiates bending of the nanopillars on the wings, causing shear stress that leads to damage of the bacterial membrane (outer and inner layers separate) and leakage of the cytoplasm. Any movement of the immobilized bacterium on the unfavorable terrain results in shear stress that first separates the bacterial outer membrane layer from the inner membrane layer and then causes subsequent cytoplasm leakage and death (Figure 3). Contrary to the commonly accepted model where nanotextured materials of uniform height are thought to be responsible for antibacterial properties, this study demonstrated that the varying heights of the nanotextured populations contribute to a shear force that results in death of bacteria adhered via EPS. The results of this study could be used to engineer nanomaterials that emulate the natural antimicrobial properties of dragonfly wings.
Nanopillars are a promising tool for microbial detection and sensing of biomolecules. Assessing microbial count on the surfaces of implants and other medical devices is a preventive measure of infections. Sensing of biomolecules in the biological system can be used to monitor the status and progression of diseases.
Sugnaux & Fischer explored bicathodic microbial fuel cell sensors with gold nanoparticles and antibiotics for the detection of microbes [10]. The antimicrobial properties of the nano-surfaces was also assessed. Microbial detection on medical implants and devices is important for preventing infections, but there is no method for quantifying the number of microbes on such objects. Nanostructured electrodes offer a method of microbial detection based on the potentiometric signals generated. The researchers of this study engineered conductive microbial fuel cells via nanoprint lithography and a templated electrodeposition technique (Figure 4). The surface topography consisted of gold nanopillars. Two gold electrode experimental models were used: one was an unstructured flat surface anode with an Au film on top, and another was nano-structured Au nanopillars. Both the samples resulted in an increasing open circuit potential at the beginning of the experiment, indicating that the E. coli bacteria had successfully attached to the sample surfaces. The flat nano-surface showed a decrease in open circuit potential over time. The nanopillar surface sample retained an open circuit potential of 0.9 V before decreasing over time as nutrients depleted. Ampicillin was added to both the nanopillar and flat Au samples, resulting in oscillatory decrease and no change in the open circuit potential, respectively. Optical microscopy confirmed bacterial adhesion was present on the nanopillar sample but not in the flat sample. The nanopillar surfaces caused low electrical currents in the current density experiments, indicating E. coli viability prior to their degradation. The electrons were confirmed to be coming from the E. coli biological system in the mediator induced power shut down experiment. Since the nanostructured gold nanopillars demonstrated bacterial viability, the researchers of this study proposed that a specific roughness at the nanoscale level is required for exploring antimicrobial properties of the nanopillars. The gold nanopillar fuel cell is a novel method for exploring microbial detection and antimicrobial activity of nanomaterials.
Pang et al. constructed electrodes made of TiO2 nanopillars (NP)/N-doped graphene quantum dots (N-GQDs)/g-C3N4 QD (TiO2 NPs/N-GQDs/g-C3N4 QDs) for the detection of hepatitis B virus (HBV) DNA for early monitoring of hepatocellular carcinoma (HCC) (Figure 5) [11]. pcDNA3 vectors were used to capture HBV DNA, forming the pcDNA3-HBV complex which was then absorbed by the TiO2 NPs/N-GQDs/g-C3N4 QDs modified electrode. The visible-light sensitive property of the biosensor allowed for improved photo-to-current transmission in order to detect low levels of pcDNA3-HBV. The authors demonstrated a decrease in photocurrent with increasing levels of pcDNA3-HBV once optimal concentrations of the biosensor components were determined. Data showed that the decreasing photocurrent was strongly proportional to the log of pcDNA3-HBV concentration (correlation coefficient 0.9949) when the viral DNA concentration ranged from 0.01 fmol/L to 20 nmol/L. Moreover, the TiO2 NPs/N-GQDs/g-C3N4 QDs biosensor displayed high sensitivity to viral DNA even at low concentrations such as 0.005 fmol/L. The TiO2 nanopillars combined with the N-GQDs and g-C3N4 QDs showed promising potential in biosensing applications.
Nanopillars have shown promising potential in biochemical studies on cell growth. The tunable dimensions of nanopillars emulate the natural extracellular matrix (ECM) and its constituents such as proteins, receptors, and other biochemical factors. Surface topography is one method to monitor cell growth and behavior for novel treatments such as induced stem cell differentiation and expression of certain cell lineages.
Migliorini et al. studied cell interaction with nanopillars to promote differentiation of embryonic stem cell-derived neuronal precursors into neuronal lineages in the absence of biochemical factors [12]. They plated embryonic neuronal stem cell precursors on polydimethylsiloxane (PDMS) nanopillars (Figure 6) to observe if the surface topography of the substrate over which the precursor cells are grown would permit neuronal differentiation in the absence of certain biochemical factors such as retinoic acid, sonic hedgehog, FGF, and other molecules. These biochemical factors were absent because in vivo biochemical-induced differentiation often leads to potential tumorigenic growth. Moreover, the optimal concentration of the factors is often difficult to regulate without potential side effects. Therefore, controlling the surface topography using nanopillars is a potential alternative for stem cell differentiation to avoid tumorigenic growth. The results showed that neuronal precursor cells plated on PMDS had higher survival rates—up to 1.9 times higher—than the same cells plated on glass coverslips. Nanoscale topography influenced the behavior of the precursors as survival was higher (2.5 times higher) and differentiation was more pronounced on the PMDS nanopillars than on the nanogrooves. Neuronal yield was nearly double on the nanopillars (60 ± 1.7) in comparison to flat PDMS (29 ± 0.2). The height of the pillar topography also determined whether the neurites grew on the top of the pillar or in more random behavior. About 40% of the neuronal precursors experienced accelerated differentiation when grown over the nanopillars. The PDMS nanopillars enhanced neuronal differentiation since they emulated the natural ECM environment the cells normally grow and differentiate in, allowing the MSC to experience extracellular cues for differentiation. The nanopillars enabled enhanced contact and interaction between the cells and the ECM. More flexible nanopillars could potentially accelerate the differentiation process as the neuronal precursors would be freer to move on top of the nanopillars, allowing for greater interaction with the medium.
Brammer et al. constructed gold-coated silicon wafers on mesenchymal stem cells (MSC) to observe how surface topography and interaction with the external cellular environment regulate growth and differentiation of the stem cells [13]. Surface topography was explored to induce differentiation of the MSC to reduce the potential side effects of biochemical induction of stem cells (Figure 7). Gold is a biocompatible metal since it does not cause major cellular damages; therefore, it was the chosen metal substrate to coat the samples. Three samples were constructed: flat Au-coated, micropillar Au-coated, and nanopillar Au-coated silicon substrates. All samples were made from gold to maintain constant surface chemistry and interaction with ECM proteins in order to observe the effects of physical features on MSC growth and differentiation. The flat and micropillar surfaces resulted in similar trends in cell adhesion and growth, but the nanopillar surface resulted in increased adhesion (at 2 h of plating) and growth (after 24 and 48 h of plating) compared to the other two samples. Moreover, the nanopillars aggregated the cells whereas the flat and micropillars did not. Differentiated cells are more likely to form aggregates with cells of the same fate based on morphological features, so the various aggregates formed in the nanopillar sample indicate that differentiation was successful with the nanopillar surface topography. Differentiation in the nanopillar sample was confirmed using alizarin red staining, which detects osteogenic mineralization, a fate of differentiating MSC. An osteoblast-specific protein, osteopontin, was also detected in high levels in the nanopillar aggregates, confirming the MSC did differentiate into the osteogenic lineage. Upregulated adhesion, growth, and aggregation using nano-structured surfaces enabled greater differentiation of the MSC into osteoblast in comparison to flat and micro-structured surfaces.
Koo et al. studied in vitro behavior of human corneal endothelial cell B4G12 (HCEC-B4G12) by altering the surface topography and biochemical cues of the microenvironment the cells were grown on [14]. Extracellular matrix proteins such as of fibronectin-collagen I (FC), FNC® coating mix (FNC), and laminin-chondroitin sulfate (LC) were coated on the surface of the fabricated PDMS micro and nanopillars. Changes in cellular morphometry, Na+/K+-ATPase and zona occludens 1 (ZO-1) gene and protein expressions were analyzed to assess the effects of the fabricated pillars on the HCEC-B4G12 growth. Circularity measurements showed that the cells grown over the pillars were highly circular, which is an important feature for assessing post-transplant corneal health. HCEC-B4G12 grown on 1 μm pillars with the FC coating resulted in the highest Na+/K+-ATPase and ZO-1 gene and protein expression for the FC-coated samples. HCEC-B4G12 grown on 250 nm pillars with LC coating resulted in the highest ZO-1 gene expression for the LC-coated samples and the lowest coefficient of variation, a parameter used to assess corneal epithelium disfunction, relative to the other patterns and the un-patterned control. This study demonstrated how the pillars served to modulate cell signaling pathways to control cell behavior and gene expression of the HCEC-B4G12 samples.
Surface topography research using nanopillars has also shown potential in studying the interactions of biomolecules with their microenvironment. Nanopillars experience various types of physical and chemical interactions with biomolecules. These studies further the understanding of the behaviors of biomolecules with surrounding substrates.
Wang et al. used nanopillar technology to study the dynamics of biomolecules at the single-molecule level [15]. The authors of this study designed nano-porous anodic alumina membranes (nanoPAAM) to fabricate poly(methylmethacrylate) (PMMA) nanopillars that were then embedded inside microchannels. Nanopillars with a diameter of 80 nm were fabricated in a highly ordered array with a distance of about 20 nm between each columnar structure. This ensured a uniform microchannel for the DNA molecules to pass through. The micro/nanostructured materials served to better demonstrate how biomolecules, such as the lambda phage DNA used, interact with their physical and chemical environment. The phage DNA was injected into the microchannel containing the nanopillar arrays. Through a combination of hydrophobic, retractive, and capillary forces, the DNA exhibited a stretching behavior, which was monitored in real-time. The nanopillar array allowed the authors to analyze the dynamics of biomolecules with their environment at the single-molecule level in a cost-effective and efficient method.
Chen et al. fabricated PMMA nanopillars with ultrahigh aspect ratios and investigated proteolytic reactions on nanotextured modified surfaces [16]. The authors reported that the Vmax of enzymatic proteolytic reactions to convert N-α-benzoyl-L-arginine-p-nitroanilide to p-nitroaniline on the PMMA nanopillar surface was roughly 10 times greater to that on planar PMMA supports. Therefore, increasing the reaction speed is important for bio-reactors in microfluidic devices.
Surface topography dynamics played by tunable nanopillars have been explored in the studies reviewed in this paper. The tunable nanopillars helped the investigation of all these studies through interactions with the biological environment at the single molecule level. The nano profile of the nanopillars allowed for enhanced interaction that emulated the natural cellular environment. Moreover, the tunable physical dimensions and chemical compositions of the nanopillars illustrated how surface topography plays a role in interactions with biomolecules, microorganisms, and the host biological system. Nanopillars are a promising tool for exploring how surface topography mechanics can be used to control biochemical outcomes of biological systems.
Nanopillars have demonstrated potential in biochemical fields studying stem cells, antimicrobial activities, biorecognition, and biomolecules. Further research on the physical and chemical properties of the nanopillars would elucidate how surface topography affects the biochemical environment and its constituents. For example, some of these studies used nanopillars constructed from gold, silicon, PMMA, and a mixture of gold and silicon. Future research can aim towards finding more biocompatible chemical compositions to not only reduce potential damage to the host biological system but to also analyze how surface topography interacts with the environment. Moreover, future studies could analyze how different sizes, shapes, and overall physical properties of the nanopillars play role in interacting with the environment. For example, antimicrobial studies could explore how nanopillars with a multitude of different heights and surface roughness affect bacterial adhesion and growth on surfaces.
The tunable dimensions and biocompatibility of nanopillars make them of particular interest in the biochemical field. The potentials of nanopillars for biochemical applications have been proven in antimicrobial, tissue engineering, biosensing, and cell culture studies. Nanopillars offer a promising potential for understanding how surface topography dynamics can be implemented for biochemical studies and engineering of antimicrobial surfaces, microbe and biomolecule detection devices, and induced differentiation of stem cells.
No conflict of interest.
[1] |
Wang EC, Wang AZ (2014) Nanoparticles and their applications in cell and molecular biology. Integr Biol 6: 9-26. doi: 10.1039/c3ib40165k
![]() |
[2] | Sathiyamoorthi P, Kim HS (2019) Nanocrystalline high entropy alloys: Processing and properties, Encyclopedia of Materials: Metals and Alloys, Elsevier, 372-380. |
[3] |
Llor C, Bjerrum L (2014) Antimicrobial resistance: risk associated with antibiotic overuse and initiatives to reduce the problem. Ther Adv Drug Saf 5: 229-241. doi: 10.1177/2042098614554919
![]() |
[4] |
Joerger RD (2003) Alternatives to antibiotics: bacteriocins, antimicrobial peptides and bacteriophages. Poultry Sci 82: 640-647. doi: 10.1093/ps/82.4.640
![]() |
[5] |
Hergt R, Hiergeist R, Hilger I, et al. (2004) Maghemite nanoparticles with very high AC-losses for application in RF-magnetic hyperthermia. J Magn Magn Mater 270: 345-357. doi: 10.1016/j.jmmm.2003.09.001
![]() |
[6] |
Gupta AK, Gupta M (2005) Synthesis and surface engineering of iron oxide nanoparticles for biomedical applications. Biomaterials 26: 3995-4021. doi: 10.1016/j.biomaterials.2004.10.012
![]() |
[7] |
Liu G, Gao J, Ai H, et al. (2013) Applications and potential toxicity of magnetic iron oxide nanoparticles. Small 9: 1533-1545. doi: 10.1002/smll.201201531
![]() |
[8] | Alhmoud H, Cifuentes-Rius A, Delalat B, et al. (2017) Gold-decorated porous silicon nanopillars for targeted hyperthermal treatment of bacterial infections. ACS Appl Mater Interf 9: 33707-33716. |
[9] |
Bandara CD, Singh S, Afara IO, et al. (2017) Bactericidal effects of natural nanotopography of dragonfly wing on Escherichia coli. ACS Appl Mater Interf 9: 6746-6760. doi: 10.1021/acsami.6b13666
![]() |
[10] |
Sugnaux M, Fischer F (2016) Biofilm vivacity and destruction on antimicrobial nanosurfaces assayed within a microbial fuel cell. Nanomed-Nanotechnol 12: 1471-1477. doi: 10.1016/j.nano.2016.03.008
![]() |
[11] |
Pang X, Bian H, Wang W, et al. (2017) A bio-chemical application of N-GQDs and g-C3N4 QDs sensitized TiO2 nanopillars for the quantitative detection of pcDNA3-HBV. Biosens Bioelectron 91: 456-464. doi: 10.1016/j.bios.2016.12.059
![]() |
[12] |
Migliorini E, Grenci G, Ban J, et al. (2011) Acceleration of neuronal precursors differentiation induced by substrate nanotopography. Biotechol Bioeng 108: 2736-2746. doi: 10.1002/bit.23232
![]() |
[13] |
Brammer KS, Choi C, Frandsen CJ, et al. (2011) Hydrophobic nanopillars initiate mesenchymal stem cell aggregation and osteo-differentiation. Acta Biomater 7: 683-90. doi: 10.1016/j.actbio.2010.09.022
![]() |
[14] |
Koo S, Muhammad R, Peh GS, et al. (2014) Micro-and nanotopography with extracellular matrix coating modulate human corneal endothelial cell behavior. Acta Biomater 10: 1975-1984. doi: 10.1016/j.actbio.2014.01.015
![]() |
[15] |
Wang K, Dang W, Xi D, et al. (2011) Hybridised functional micro-and nanostructure for studying the kinetics of a single biomolecule. Micro Nano Lett 6: 292-295. doi: 10.1049/mnl.2010.0215
![]() |
[16] |
Chen G, McCarley RL, Soper SA, et al. (2007) Functional template-derived poly(methyl methacrylate) nanopillars for solid-phase biological reactions. Chem Mater 19: 3855-3857. doi: 10.1021/cm0702870
![]() |
1. | Bernd Schmidt, Jiří Zeman, A Bending-Torsion Theory for Thin and Ultrathin Rods as a \(\boldsymbol{\Gamma}\)-Limit of Atomistic Models, 2023, 21, 1540-3459, 1717, 10.1137/22M1517640 |