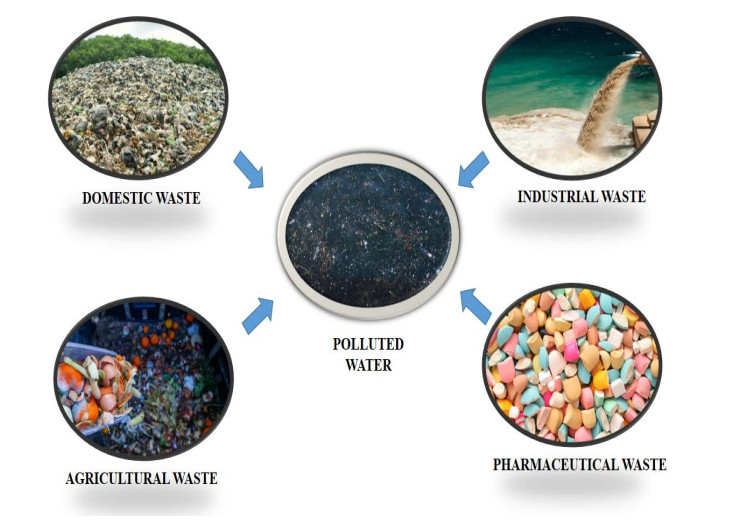
In this review, we describe recent developments and strategies involved in the utilization of solid supports for the management of wastewater by means of biological treatments. The origin of wastewater determines whether it is considered natural or industrial waste, and the source(s) singly or collectively contribute to increase water pollution. Pollution is a threat to aquatic and humans; thus, before the discharge of treated waters back into the environment, wastewater is put through a number of treatment processes to ensure its safety for human use. Biological treatment or bioremediation has become increasingly popular due to its positive impact on the ecosystem, high level of productivity, and process application cost-effectiveness. Bioremediation involving the use of microbial cell immobilization has demonstrated enhanced effectiveness compared to free cells. This constitutes a significant departure from traditional bioremediation practices (entrapment, adsorption, encapsulation), in addition to its ability to engage in covalent bonding and cross-linking. Thus, we took a comparative look at the existing and emerging immobilization methods and the related challenges, focusing on the future. Furthermore, our work stands out by highlighting emerging state-of-the-art tools that are bioinspired [enzymes, reactive permeable barriers linked to electrokinetic, magnetic cross-linked enzyme aggregates (CLEAs), bio-coated films, microbiocenosis], as well as the use of nanosized biochar and engineered cells or their bioproducts targeted at enhancing the removal efficiency of metals, carbonates, organic matter, and other toxicants and pollutants. The potential integration of 'omics' technologies for enhancing and revealing new insights into bioremediation via cell immobilization is also discussed.
Citation: Frank Abimbola Ogundolie, Olorunfemi Oyewole Babalola, Charles Oluwaseun Adetunji, Christiana Eleojo Aruwa, Jacqueline Njikam Manjia, Taoheed Kolawole Muftaudeen. A review on bioremediation by microbial immobilization-an effective alternative for wastewater treatment[J]. AIMS Environmental Science, 2024, 11(6): 918-939. doi: 10.3934/environsci.2024046
[1] | Joan Nyika, Megersa Olumana Dinka . A mini-review on wastewater treatment through bioremediation towards enhanced field applications of the technology. AIMS Environmental Science, 2022, 9(4): 403-431. doi: 10.3934/environsci.2022025 |
[2] | Abigail W. Porter, Sarah J. Wolfson, Lily. Young . Pharmaceutical transforming microbes from wastewater and natural environments can colonize microplastics. AIMS Environmental Science, 2020, 7(1): 99-116. doi: 10.3934/environsci.2020006 |
[3] | Rafael Gonzalez-Olmos . Leading edge technologies in wastewater treatment. AIMS Environmental Science, 2015, 2(2): 237-240. doi: 10.3934/environsci.2015.2.237 |
[4] | Ankit Srivastava, T.C. Prathna . Urban water resource management: experience from the revival of Rajokri lake in Delhi. AIMS Environmental Science, 2021, 8(5): 421-434. doi: 10.3934/environsci.2021027 |
[5] | Jianfeng Wen, Yanjin Liu, Yunjie Tu and Mark W. LeChevallier . Energy and chemical efficient nitrogen removal at a full-scale MBR water reuse facility. AIMS Environmental Science, 2015, 2(1): 42-55. doi: 10.3934/environsci.2015.1.42 |
[6] | Volodymyr Ivanov, Viktor Stabnikov, Chen Hong Guo, Olena Stabnikova, Zubair Ahmed, In S. Kim, and Eng-Ban Shuy . Wastewater engineering applications of BioIronTech process based on the biogeochemical cycle of iron bioreduction and (bio)oxidation. AIMS Environmental Science, 2014, 1(2): 53-66. doi: 10.3934/environsci.2014.2.53 |
[7] | Liron Shoshani, Asher Brenner, Chaim Sheindorf . Use of an integrated biophysical process for the treatment of halo- and nitro- organic wastes. AIMS Environmental Science, 2017, 4(4): 523-539. doi: 10.3934/environsci.2017.4.523 |
[8] | Gireshsingh Mungla, Sunita Facknath, Bhanooduth Lalljee . Assessing the Potential of Mechanical Aeration Combined with Bioremediation Process in Soils and Coastal Sediments Impacted by Heavy Metals. AIMS Environmental Science, 2022, 9(5): 692-707. doi: 10.3934/environsci.2022039 |
[9] | Melanie Voigt, Alexander Wirtz, Kerstin Hoffmann-Jacobsen, Martin Jaeger . Prior art for the development of a fourth purification stage in wastewater treatment plant for the elimination of anthropogenic micropollutants-a short-review. AIMS Environmental Science, 2020, 7(1): 69-98. doi: 10.3934/environsci.2020005 |
[10] | Cornelius Tsamo, Tita Mangi Germaine, Adjia Henriette Zangue . Reuse of wastewaters from slaughterhouse and palm oil mill: Influence on the growth performance of catfish (Clarias gariepinus). AIMS Environmental Science, 2023, 10(6): 743-763. doi: 10.3934/environsci.2023041 |
In this review, we describe recent developments and strategies involved in the utilization of solid supports for the management of wastewater by means of biological treatments. The origin of wastewater determines whether it is considered natural or industrial waste, and the source(s) singly or collectively contribute to increase water pollution. Pollution is a threat to aquatic and humans; thus, before the discharge of treated waters back into the environment, wastewater is put through a number of treatment processes to ensure its safety for human use. Biological treatment or bioremediation has become increasingly popular due to its positive impact on the ecosystem, high level of productivity, and process application cost-effectiveness. Bioremediation involving the use of microbial cell immobilization has demonstrated enhanced effectiveness compared to free cells. This constitutes a significant departure from traditional bioremediation practices (entrapment, adsorption, encapsulation), in addition to its ability to engage in covalent bonding and cross-linking. Thus, we took a comparative look at the existing and emerging immobilization methods and the related challenges, focusing on the future. Furthermore, our work stands out by highlighting emerging state-of-the-art tools that are bioinspired [enzymes, reactive permeable barriers linked to electrokinetic, magnetic cross-linked enzyme aggregates (CLEAs), bio-coated films, microbiocenosis], as well as the use of nanosized biochar and engineered cells or their bioproducts targeted at enhancing the removal efficiency of metals, carbonates, organic matter, and other toxicants and pollutants. The potential integration of 'omics' technologies for enhancing and revealing new insights into bioremediation via cell immobilization is also discussed.
Water is the most abundant odorless and colorless liquid in the world. It is essential to the survival of all living things and accounts for approximately 71% of the earth's surface. Its use ranges from domestic to agricultural, industrial, and even automotive, among others. Nonetheless, water becomes polluted when unwanted materials or contaminants such as industrial (sludges) and agricultural wastes, pesticides, and herbicides run off, and toxic organic or inorganic chemicals enter water bodies [1,2]. Natural disasters such as earthquakes, tsunamis, hurricanes, volcanoes, and floods also contaminate water bodies. Pollutants have the potential to reduce the quality of available environmentally wholesome water. Pollution poses a significant threat to human life, particularly when water is used for other non-industrial purposes such as drinking and bathing, thus predisposing people to diseases like cholera, typhoid fever, and tuberculosis. As such, a close association exists between polluted water use or consumption and water-borne diseases [3,4,5,6,7,8].
Due to rapid economic growth and industrialization, regions like the Asian continent (China now experience significant environmental degradation directly tied to industrial processes. In addition to causing significant ecological damage, pollutants are responsible for eutrophication and widespread organic and toxic pollution [4]. Additionally, due to the direct consequences of polluted water [9,10,11,12], many people are at risk of developing cancer-related diseases. Owing to this, industries have been prompted to look for suitable wastewater treatment systems in addition to strengthening and enforcing stringent regulatory requirements [13].
Different processes (physical, chemical, biological) are employed to purify water and wastewater, some of which include membrane processes, osmosis, foam flotation, coagulation, dialysis, adsorption, biological methods, and photocatalytic degradation. However, their application is limited due to various factors, such as engineering expertise, energy requirements, economic implication, processing efficiency, and infrastructure. These factors, along with others, hinder their universal acceptance and utilization. The conventional treatment of wastewater is not always capable of removing the entire contaminant load because of the complexity of the chemical mixtures that are found in wastewater. This is because of the fact that conventional wastewater treatment relies on biological processes [2,14]. The immobilization of viable cells constitutes a promising method for treating wastewater in a manner that is environmentally friendly. According to the findings of Xiaofan [15], refractory organics were effectively reduced by utilizing immobilized microbial technology to obtain cultured bacteria relative to fixing on a carrier in order to obtain maximum production of enzymes from the microbial cells. This allows an increase in the number of microbes capable of degrading the refractory organics.
In addition, several technologies continue to emerge and could guide the future of research targeted at the remediation of pollutants via the use of immobilized microbial cells. Some of these tools and workflows are 'omics'-based [16]. Other technologies are based on single cells, whole cells or their products, and hence largely bioinspired. Some examples of these include emergent tools include enzymes immobilization, biological permeable reactive barriers linked to electrokinetic tools [17,18], magnetic cross-linked enzyme aggregates (CLEAs), thin-filmed bio-coatings, encouraging the formation of microbiocenosis using Vija fibrous barrier [19], nanotechnology inspired nanosized biochar, and engineering of select microbial strains or their products [20]. The single use or integration of these techniques are generally aimed at enhancing the bioremediation efficacy xenobiotics, harmful phenolic compounds, carbonates, organic matter, heavy metals, among other pollutants that pose health risks to humans and animals. The potential integration of 'omics' technologies for enhancing and revealing new insights into bioremediation via cell immobilization is also discussed.
In light of the above, we therefore focus on the immobilization of microorganisms for the biodegradation of water pollutants. We provide an overview of the available, as well as emerging, immobilization technological trends and the associated challenges from a futuristic standpoint. The work also differs from others published [21,22] on the topic by its incorporation of discussion on state-of-the-art tools that are bioinspired, involve engineered microbes and 'omics' tools, among others, in a bid to significantly improve the remediation efficiency and ability of wastewater treatment processes to mop-up pollutants.
What is understood as pollution is the act of introducing a contaminant into an environment [1,2]. Through a process referred to as water pollution, contaminants such as human waste, sewage, waste from industrial processes, toxic chemicals, and other potentially hazardous components could make their way into bodies of water (Figure 1). As a direct consequence of this, the water becomes contaminated and unsuitable for drinking by humans [23].
Naturally, rainfall contributes to water pollution when rainwater runs off into a water body. Anthropogenic factors and industrialization are other potential contributors to the deterioration of water quality. Insecticides, fluoride, heavy metals, pesticides, dyes, phenols, personal care products (PCPs), and pharmaceuticals, as well as fluoride (Figure 1) also pollute water bodies [24]. The level of water contamination increases in proportion to thehuman population in a given area. The primary contributors to pollution in aquatic habitats are the byproducts of agriculture, domestic, and industrial activities, for example, sewage dumped into lakes, rivers, or other bodies of fresh water [1,25,26].
Industrial wastes, for example, polycyclic aromatic hydrocarbons (PAHs), petrochemicals, heavy metals, pesticides, phenolic compounds, polychlorinated biphenyls (PCBs), microbes, dioxins, and phenolic compounds are major causes of water pollution. Their continued discharge into aquatic bodies has long-term consequences on the environment, affecting food availability and posing a serious threat to aquatic life. These pollutants are typically released into bodies of water without treatment, and the cumulative detrimental impacts on the ecosystem have garnered much attention. Some of the wastes, especially heavy metals present in this wastewater, are carcinogenic, while others are poisonous to the aquatic ecosystem [27,28]. According to Begum [29], cobalt (Co), nickel (Ni), manganese (Mn), zinc (Zn), chromium (Cr), lead (Pb), and copper (Cu) are heavy metals that have been found in Indian's Cauvery River as a result of the industrial, anthropogenic and agricultural activities near the river. This is in congruence with the report by Sunita [30], who reported high levels of chromium, lead, zinc, and several other metals in samples collected from the Yamuna River in Delhi, India, indicating serious toxicity to aquatic life and adverse bio-magnification effect to humans [29,30].
The infrastructures needed for the treatment of wastewater in villages, small towns, and some metropolitan areas is practically unavailable. Domestic garbage is often emptied into local river channels connected to large water bodies. Household sewage is also often dumped directly into rivers in metropolitan areas. Solid trash and domestic waste are also not properly disposed of but stacked up and buried without permission. These acts enhance water pollution, especially during rainy seasons [31,32,33]. Due to the large volume of untreated effluents from industries and urban areas, the receiving water bodies become heavily polluted. Similarly, Swapnil [34] reported the disposal of huge amounts of domestic wastes into the Panchaganga water body from Kolhapur (India) city, which resulted in an increased level of organic matter polluting the water body. This was characterized especially by plastic litter, toxicants, solid wastes, and microbial contaminants [34]. In essence, human fecal and residential wastes discharged into the river, rather than into sewage treatment plants, constitute the likely source of enteric pathogen pollution [35].
The excessive and continuous usage of agrochemicals in riverine areas, flooding, high rains, and excessive irrigation, among other factors, ensure pesticides and chemical residues are leached into rivers, thereby entering the food chain [34,36]. As a result of dietary changes, the increased demand for poultry meat products has increased wastewater from poultry and livestock industries, and this forms another source of water pollution [32]. Not only that, animal excrement in agricultural areas have also contributed to eutrophication and pollution as pathogens, organics, and nutrient runoff during rainfalls enter water bodies [37].
A variety of pharmaceutical chemicals employed in processes in the industry are highly toxic when released into the environment and may either directly or indirectly cause oxidative changes. Pharmaceutical companies generate a lot of trash during manufacturing and maintenance processes, and some of the debris finds its way into wastewater treatment plant effluents, as well as portable water sources. Little amounts of drug particles in these water sources could also pose serious health hazards to human and aquatic ecosystems [38,39]. With respect to a recently concluded study on contaminants in the river basin, active pharmaceutical ingredients commonly used in human treatment contaminate large volumes of groundwater and surface waters [40,41].
Decontamination becomes necessary in response to the negative effects of wastewater in the environment. As cities grow in size, accessible land for wastewater treatment and disposal shriveled. Wastewater generation increased rapidly with population growth, reducing water quality by outpacing streams and rivers' self-purification capacities [42,43]. In Table 1, the purification process is summarized into four major phases.
S/N | Methods | Treatments |
1 | Preliminary treatment | Removing large materials/solids in wastewater, such as cloth, paper, wood, garbage, plastics, feces, metal or glass, and gravel. |
2 | Primary treatment | Physical processes, floatation and sedimentation techniques are used in removing organic and inorganic solids, oils as well as grease. |
3 | Secondary treatment | Confiscating the remaining organic matter and suspended solids through biological treatment using a variety of microorganisms. |
4 | Advanced treatment |
Physicochemical treatment, tertiary treatment, and combined biological-physical treatments. It entails the reduction of nitrogenous oxygen demand (NOD), nutrient extraction, and more toxic substances. |
The immobilization of microbial cells as a treatment method has recently gained traction in the field of waste management. In comparison to more conventional systems, the cell immobilization technology offers a significant benefit [44,45]. The process of cell immobilization involves the localization of microbial cells to a specific space. This is done to limit the cell's ability to freely migrate, to exhibit hydrodynamic properties that are distinct from those of the surrounding environment, and to keep the cell's catalytic activities intact for continued and repeated use. Immobilized cells have been investigated as a potential alternative technology for applications in environmental-related research and has gained traction not only in industrial but in agriculture, biocontrol, pesticides, and in biodegradation of soil pollutants as well as contaminated groundwater [44,45]. Microorganisms preserved on a carrier can be used in production processes that are either continuous or semi-continuous, which results in significant cost savings because biocatalysts do not require any sort of replenishment for optimal functioning [46,47,48]. Immobilized microorganisms, due to their high microbial density, rapid response, resistance to external impact, low sludge production, and easy-to-control advantages, naturally break down and cleanse wastewater off organic compound. Furthermore, the cost of biological techniques is noticeably lower than that of physical or chemical means [2,49].
There are four (4) widely adopted approaches in cell immobilization, as demonstrated in Figure 2. Physical adsorption is the most economical and cost-effective while cross-linking or covalent bonds are the most expensive [21]. Entrapment and encapsulation fall in between, with encapsulation potentially having higher long-term costs due to its complexity [50]. Physical adsorption and encapsulation generally have low to moderate risks of secondary pollution. Cross-linking or covalent bonds offer minimal risk, whereas entrapment could pose moderate risks if the matrix degrades. Cross-linking or covalent bonds and encapsulation are the most effective in terms of stability and containment. Physical adsorption and entrapment are also effective but can be limited by factors like saturation and degradation [21,51]. The methods are further discussed in the proceeding subsections. Furthermore, in a bid to give a comprehensive overview for ease of comprehension, the pros and cons associated with each of these methods are summarized in Table 2 for ease of comprehension.
Methods | Economy | Effectiveness | Durability | Secondary Pollution | References |
Entrapment | Moderate to high Cost | Effective for retaining substances within a matrix | Slightly durable and requires higher cost of maintenance | Moderate form of pollution which occurs only when matrix degrades | [50;52,53,54,55] |
Physical adsorption | • Inexpensive due to low cost of material and processes • Suitable for many pollutants • Absorbents can be regenerated and reused |
• Moderately effective • Efficiency can be reduced due to saturation of adsorbents • This method can be less effective if the pollutants involved have high specificity |
Very durable | There is minimal risk of secondary pollution | [21] [54,55,56] |
Cross-Linking or covalent Bonds | • High cost due to complex processes and expensive chemicals. • The most expensive method of immobilization. • Though this the most expensive method, but its durability justifies its cost for long term applications. |
• Highly effective for both short- and long-term applications • Most effective for tailored applications and/ or contaminants |
Most durable solution amongst these four methods of immobilization | • Risk of secondary pollution is very low due to the stability of covalent bonds • Cross-linking agents can be toxic if degraded |
[22,57,58] |
Encapsulation | • Cost of production is moderate and it can be high depending on the material and technology used for encapsulation • More expensive when compared to physical adsorption method |
• Effectiveness of this method is high • Very useful in preventing the release of contaminants. • This method can also be designed for various applications • They can also be designed for specific applications and/or contaminants |
• Materials used in this method has a longer lifespan • This reduces the long-term costs. |
• Low and can be moderate • Improper encapsulation can lead to higher risks • Leaching of polymeric gels or other encapsulation materials can pose great risk |
[21,51,56,59,60] |
In this technique, it is possible for hydrogen bonding, dipole-dipole interactions, van der Waals forces, or hydrophobic interactions to take place during the process of absorption. The properties of both the substrate and the adsorbate determine which interaction is employed for immobilization. Physical adsorption is favored for its simplicity, ease of cell desorption enhancing reusability, minimal interference on microbial growth, flexibility, cost-effectiveness (economical for large-scale wastewater treatment), and use of a wide range of available solid supports or carriers (activated carbon, silica, resins) selected based on the specific conditions of the wastewater [44,45]. Nevertheless, a major drawback of this method is that weak forces are used for cell attachment and could be insufficient in dynamic environments (high agitation and flow rate), such that cell desorption occurs. Other challenges include the potential for non-specific binding, shorter operational lifespan, and sensitivity to abiotic factors (pH, ionic strength, temperature), all of which contribute to biocatalytic activity loss and reduced immobilization efficiency [61]. From example, A study immobilized laccase on an inexpensive nanosized bagasse magnetic biochar via adsorption, precipitation (glutaraldehyde and ammonium sulfate), and cross-linking agents. The biochar carrier enhanced enzyme immobilization, increasing catalytic activity, storage stability, and tolerance to temperature and pH flux. Yet, the immobilized laccase catalytic action was reduced to more than 50% at pH of 5.5 and 6, thus indicating the method's susceptibility to certain prevailing environmental conditions [20].
The entrapment technique involves the placement of immobilized cells in fibers or a support matrix and then encasing the entire structure in a matrix of either collagen, alginate, agar, carrageenan, cellulose, gelatin, polyacrylamide, polystyrene, epoxy resin, polyester, polyurethane, and/or photo cross-linkable resins during this irreversible process [44]. This method has garnered the most attention from researchers because it results in the formation of a defensive shield around the immobilized microbes. This not only ensures the microbe(s) continuous viability throughout the treatment processing step but also maintains their viability while in the polymer storage matrix [62,63,64]. Furthermore, entrapment minimizes cell leakage, ensuring that immobilized cells remain within the matrix throughout the bioremediation process [65]. Its major benefits are the robust protection to cells, high suitability to harsh industrial conditions or toxicant and extreme environmental shifts, and its operational and mechanical stability for treatment over long periods. Matrices may also be specifically engineered to be selectively permeable to enhance penetration of certain substrates and removal of pollutants [66]. On the other hand, its key disadvantage lies in the entrapment matrix inhibiting nitrogen, gas (oxygen), and substrates diffusion to immobilized cells, thus negatively impacting bioremediation efficiency. Its scale-up could also be quite a challenge as uniformity in cell distribution and matrix integrity must be maintained. Maintaining these two key factors increases operational costs and suitability for industry-scale use. Added drawbacks include the potential to form biofilms on matrix surfaces, matric degradation over time (reduced reusability), process clogging, and decrease efficiency [67,68]. A study has shown that creating magnetic cross-linked enzyme aggregates (CLEAs) or their entrapment in particles with improved mechanical characteristics enhances enzyme immobilization, even with the addition of bovine serum albumin (BSA), 3-aminopropyltriethoxysilane (APTES), glutaraldehyde (cross-linker), and ammonium sulfate (precipitant). Again, the authors showed that substrate diffusion challenges could be averted by increasing CLEAs porosity. The dense structure of CLEAs, which may impede substrate movement and lower efficiency, posed a challenge for diffusion limitations even with these advancements [69].
Encapsulation is another non-reversible method of immobilization that works similarly to entrapment in that it encloses the target. During this process, the biocatalysts are confined by the membrane walls (which typically take the form of a capsule), but they are permitted to freely move around within the core space of the cell. A biocatalyst cell has a semi-permeable membrane that allows some molecules to pass through. This enables the flow of substrates, as well as nutrients, while maintaining the integrity of the biocatalyst's location inside the cell. This phenomenon (permeability), however, depends on the membrane pore size, and is proportional to the core material size. The most significant benefit of this method is the microencapsulation which prevents access to the capsule's interior thereby protecting the biocatalyst from harsh environmental conditions [44,62]. The benefits of this methods include the immobilization a large number of cells within a small volume, good shielding of cells from harsh abiotic factors, its ability to offer controlled release of enzymes or cells, all contributing to extend operational lifespan and enhance bioremediation efficiency [70]. The drawbacks of this method include immobilization process complexity (multi-stepped emulsification, gelation), poor cell recovery rate, mass transfer barrier creation (encapsulating material could inhibit diffusion of essential substrates or oxygen), thus increasing cost and reducing treatment efficiency and industry-scale use [70]. A study demonstrated that incorporating silica into alginate significantly improved laccase encapsulation efficiency to 70%, relative to 59% when alginate was used alone. Again, laccase extract encapsulation in silica sol-gel enhanced catalytic activity and efficiency of the immobilized enzyme compared to its free form (kcat of 89.9 for alginate, 63.7 for alginate-silica, and kcat of 56.9 min−1 for silica sol-gel). Nevertheless, following use in 3 reaction cycles, the catalytic action of the enzyme encapsulated in alginate-silica was reduced by 50%. As such, this technique is limited with regards to the reuse of the enzyme in multiple reaction cycles [71].
This method is characterized by the formation of bonds (covalent) between the solid support and the cell. The method requires a binding agent (crosslinker) for this mechanism to take place. A surface must first go through the process of chemical modification in order for covalent bonds to be formed. Although the method is efficient and durable for microbial products (enzymes), it is rarely used to immobilize cells because covalent bonding agents are typically cytotoxic and hardly can cells be immobilized without being damaged [64]. Although successful covalent immobilization in yeast is not very common, Navarro and Durand [72] reported that Saccharomyces carlsbergensis successfully bonded to porous silica beads covalently. In addition, the benefits of this technique include long-term maintenance of biocatalytic activity, and reduction in cell leakage (duet to strength of bonds formed), all factors that are integral to ensuring continuous wastewater treatment and improved operational efficiency. According to Górecka and Jastrzębska [62] and Bouabidi et al. [56], covalent bonds improve immobilized cells' resilience against harsh abiotic conditions and toxic compounds. However, the method's drawbacks include the harmful nature cross-linkers used (carbodiimide, glutaraldehyde), which may reduce cell metabolism and viability, this adversely impacting the remediation process and increasing operating costs. Cross-linking is also an irreversible process, making cell recovery difficult [73]. The techniques may also not be suited to wastewater types containing high levels of organic matter/compounds, which interfere with the bonding process. The use of multipoint covalent attachment to support surfaces via use of cyanates, tosyl chloride, N-hydroxy-succinimide esters (conventional carriers) with polyethylene glycol (PEG) as a modifying agent has been investigated. Its ability to form multiple bonds between the enzyme and the support is advantageous to stabilizing the enzyme by preventing structural changes caused by heat and organic cosolvents. Yet, achieving results that were reproducibility remained a major challenge, particularly with ensuring the desired bonding parameters are maintained [74]. Hence, to enhance results reliability, cell reusability, and retention of activities, further optimization studies are required.
An emerging trend is the increasing use of microbial consortia in wastewater treatment to achieve enhanced ecological sustainability, pollutants removal. An algae-bacteria consortium may be applied to optimize wastewater treatment [75]. Likewise, the use of transformed or engineered microbial strains with highly developed detoxification mechanisms could immensely help assuage the current threat of wastewater. Nevertheless, participating consortiums must be kept stable. This is a drawback that has to be circumvented alongside the selection of strains, systems modeling, and operational parameters optimizing. Along the same lines, the generation of commercially viable and eco-friendly technological options cannot be overemphasized. Likewise, to achieve significant breakthroughs for wastewater remediation, it is essential that engineered microbes be integrated from start to finish, that is, from laboratory to pilot and industry scales [76].
In another technique, Wang et al. [17] proposed a new electrokinetic (EK)-mediated bioremediation for the removal of lead and copper heavy metals and ammonia. However, it also involved the deployment of a biological permeable reactive barrier halfway through the reaction. This facilitated the mop-up of 34–36% of the heavy metals, while the total removal of ammonia was achieved. As such, this method could be adapted for the reversal of eutrophication and clean-up of lead and copper from other hydro ecosystems such as wastewater. In a related work by these authors, the addition of ethylenediamine disuccinic acid and struvite showed the potential to enhance the removal of the same metals in the EK-bio-reactive barrier technology [18].
In addition, biological coatings have been reported to have the potential to steer environmental biotechnologies in new future directions. such as wastewater bioremediation [77]. Biosensing bio-coated films (less than 10 μm in thickness) have been shown to selectively and rapidly detect low amounts of pollutants, gases, and other toxicants in water and wastewater. Besides being robust, these coatings have the added advantage of high reactivity relative to biosensors made of whole cells [78]. These films have been formulated into microbial inks that dry fast via 3D printers [79]. Cells entrapped in bio-coated films may also be used as stable, ready-to-use agents with long shelf life, and upon activation constitute highly reactive and reproducible biosensing cells. The stabilization of fast-drying bio-coats using osmo-protectants (carbohydrates) via ink-jet printers, high-speed coating techniques have also been reported [80].
The use of biological coatings enhances the efficiency of mass transfer challenges, for example, by ensuring adequate illumination of highly concentrated photo-reactive algae or bacterial cells entrapped in thin liquid films. Again, for all immobilization techniques, the formation of biofilms is a key limitation. Nevertheless, bioengineering cells to improve their resistance and tolerance capabilities, reveal novel metabolic pathways for non-viable cells and interaction engineering (cell surface to adhesive polymer binders) remain key challenges to advancing future uses of bio-coating films in bioremediation of wastewater. It is expected that further developments from integrated sciences such as coating technologies, synthetic biology, nanomaterials, nanoscience would generate new technologies for the creation of new functional systems for cell stabilization, for example, low-cost, high intensity, potential, and efficacious engineered whole cells in bio-coatings for remediation of wastewater [77]. Furthermore, the use 3D printed biofilm carriers has demonstrated stronger adhesion and activity features by yielding almost ideal mass transfer and surface characteristics [81].
Furthermore, since immobilization techniques border around the concepts of cellular bioaccumulation, complexation, biosorption, and precipitation, several 'omics' tools (proteomics, metabolomics, transcriptomics, metagenomics) could be used to reveal secretion and functional roles of immobilized microbes in wastewater treatment plants and environmental samples, relative to the techniques used in immobilizing the cells [82]. While fluxomics could enhance our comprehension of metabolic rates of immobilized cells used in the remediation of samples [83], metabolomics could give insights into the identity and quantity of metabolic byproducts released into an environment before and after wastewater treatment [84]. In addition, for the mop-up of harmful phenolics from wastewater, immobilized oxidoreductases have proved to be promising biocatalytic tools for water purification [85]. The use of the fibrous material (Vija) as a carrier for the formation of immobilized microbiocenosis (interacting group of microbes from a habitat) has also proved effective for petroleum products removal from wastewater [16,19].
Overall, despite the potential of each technique, microbial immobilization technologies face several challenges that need to be addressed to improve effectiveness and applicability in environmental remediation. These challenges include maintaining microbial viability, selecting appropriate matrix materials, scaling up from laboratory to field applications, managing costs, addressing environmental and safety concerns, bridging technological and knowledge gaps, navigating regulatory issues, and implementing effective performance monitoring. Addressing these challenges through research, innovation, and development remains crucial to advancing these technologies and expanding their use in environmental protection.
Wastewater treatment removes chemical or biological impurities from impure water sources, such as home, industrial, or agricultural waste. This could be achieved using physical and biological processes. The liquid wastes released into the environment by households, industrial operations, or agricultural practices are among water contamination sources. Conventional wastewater treatment technologies such as filtration, sedimentation, flocculation, chlorination, and activated sludges are broken down into three categories; physical, chemical, and biological treatment. However, these methods focus on the isolation or removal of pollutants from wastewater. Subsequent steps are required in order to get rid of the contaminants that were isolated in the previous step. As a result, the development of an integrated method for treating wastewater is required and should be one that is able to remove undesirable components from wastewater while simultaneously transforming components into valuable products. Using a particular immobilization process or coupling one or more methods it is possible to accomplish this goal successfully [86,87].
In a study, three different bacterial consortia strains were immobilized on a novel biofilm and allowed to grow in order to treat wastewater that contained acetonitrile. Within a 24 h period, the biofilm reactor showed high resilience to acetonitrile loading and depleted the initial concentration of acetonitrile. According to the findings, bacteria that were immobilized on biofilm improved pollutant degradation in wastewater. These bacteria have been tested in a laboratory setting and demonstrated that the method is effective in improving the oxidation of toxicants in wastewater [88].
The efficiency of conventional biological treatment methods is diminished when it comes to cleaning up wastewater that contains refractory organics such as cyanide, aniline, and phenol, among other pollutants. This is because microorganisms need a longer period of time to complete their life cycles and have a difficult time surviving in conventional biological treatment structures. However, through immobilization technology, bacterial cultures at the highest concentration were identified and attached to the carrier, thus boosting microbial culture concentration. This effectively dealt with volatile organic compounds. In order to immobilize Fusarium species and allow for the degradation of phenols in a complete mixer, polyurethane foam was developed as a carrier. Carrageenan, alginate, agar, and polyacrylamide were investigated as potential carriers of immobilized microorganisms for the degradation of phenol by Xiaofan et al. [15]. Phenol degradation via immobilized microorganisms was significantly faster due to their access to a greater number of phenol molecules compared to free cells despite the immobilized microorganisms having a lower growth rate [89].
When treating high concentrations of organic wastewater, the technology that uses immobilized microorganisms shows relatively high treatment efficiency. This is because immobilized microorganisms are more effective at breaking down organic matter. Polyvinyl alcohol (PVA) encapsulation by a bacterial consortium was performed by Liu et al. [90] in order to treat wastewater and remove inherent dyes. The rate of decolorization was relatively stable at about 85% without any problems occurring. After operating continuously for a whole month, the rate of decolorization was in the 70 to 80% range.
It is widely believed that microbes must first go through aerobic nitrification before anaerobic denitrification in order to successfully remove ammonia and nitrogen from the environment. Cao Guomin et al. [91] developed a new method of biological denitrification that only requires a single stage and used PVA as the carrier. Using an encapsulated membrane on both sides, nitrifying bacteria oxidized ammonia to nitrite, and the same film was used by denitrifying bacteria to reduce nitrate to nitrogen. This procedure is carried out repeatedly. Once this process has been repeated several times, the ammonia will eventually be converted into nitrogen. In addition, since algae are able to bioaccumulate metal ions, they may be used to remove toxic or radioactive metals and recycle rare or precious metals in industrial wastewater. In a related study, the rate at which immobilized cells absorbed copper (II) from water was noticeably higher than the rate at which un-immobilized cells did. Likewise, immobilized algal cells have been used for desorption and regeneration at a rate of 0.5 mol/L, and their desorption rate was higher than 80% [15].
Support matrices used for immobilization could be expensive, so it is imperative to choose economical and eco-friendly ones. Low-cost matrices such as bagasse, coconut coir, loofah sponge, and gunny bag (jute) have been used to effectively immobilize Aspergillus terreus [88]. After being immobilized on the matrices listed, A. terreus significantly decreased biological oxygen demand (BOD) and chemical oxygen demand (COD), both key parameters that measure oxygen utilization by microorganisms in wastewater. When compared to other matrices, gunny bags proved to be the most effective immobilizing material for A. terreus with respect to reducing levels of pollution and improving the quality of effluent. Consequently, it was reported that the A. terreus strain was useful for the removal of dyes and degradation of organic wastes in effluents [92].
The advantages of utilizing different approaches of cell immobilization when applied to bioreactor systems have been reported. Encapsulating cells in a polymer gel matrix have proved to be successful in the laboratory and in commercial applications. It was demonstrated in bioreactors that the performance of encapsulated cells was better than free cells across a broad spectrum of environmental parameters. Encapsulated cells, for example, have higher levels of metabolic activity and metabolite production in addition to higher plasmid stability when compared to free cells. This is because plasmids are more protected within the encapsulation, which increases the level of protection afforded to plasmids [45].
Immobilizing a number of cell cultures using an immobilization matrix serves multiple purposes. For example, in the production of dihydroxyacetone in a continuous fashion, Chlorella pyrenoidosa and Gluconobacter oxydans (an algae and bacteria, respectively) were co-immobilized in calcium alginate beads [93]. During the first six days of the bioprocess, there was no significant drop in the activity levels. Upon the conversion of glycerol to dihydroxyacetone, algal cells were used as an in-situ oxygen source [93], converting glycerol into dihydroxyacetone more quickly. Another study investigated whether or not the microalgae Scenedesmus obliquus, and Bacillus subtilis bacterium could be co-immobilized in carrageenan beads and placed inside air-lift reactors in order to increase the amount of alpha-amylase enzyme production. In order to complete their processes, the bacterial cells required the assistance of the microalgal cells. The bacterial cells were primarily accountable for the production of the alpha-amylase enzyme (Table 3). By utilizing the microalgal cells as a source of oxygen production in the field, this objective was successfully accomplished. Co-immobilization was the solution to the problems that were taking place with oxygen diffusion, and as a consequence, it resulted in an increase in alpha-amylase activity that was approximately twenty percent higher. It was also discovered that when algal cells were co-immobilized with bacteria, they grew faster than when the algal cells were immobilized alone [94]. This was discovered when they compared the two methods of immobilization. Again, Dunaliella tertiolecta encapsulation in alginate and Dunaliella salina encapsulation in agar-agar, both green algae, enhanced glycerol production [88]. In a similar development, Wikstrom et al. used immobilized algae to produce keto acids from amino acids [95].
Microorganism | Polymer Type | Application | Reference(s) |
Bacillus spp., Rhodococcus spp. | Polyvinyl alcohol (PVA) cryogel | Removal of ammonia | [96,97] |
Actinobacillus succinogenes, Rhizopus oryzae spp. | PVA cryogel | Succinic, fumaric, as well as lactic acid removal | [56] |
Aspergillus awamori | Polyacrylonitrile membrane | Phenol biodegradation | [98] |
Bacillus cereus | Polyacrylamide (PAAam)- N, N'-methylenebisacrylamide (BisAAm) cryogel | Degradation of crude oil | [99] |
Bacillus spp. | PVA- H3BO3-Ca- Alg beads | Removal of PAH | [100] |
Chlorella spp. | Agar beads, agarose, carrageenan, polyurethane foam and alginate | Removes ions Zn, Pb, Ni, Cu, Cd, Hg, nitrite, NH4 and uranium |
[101,102,103,104] |
Chlorella spp., Phormidium spp., Scenedesmus obliquus, Stichococcus spp., | Capron fibers (synthetic) | Removes oil spill, heavy metals, and phenols | [105] |
Phormidium laminosum | Epoxy resin as well as polysulphone | Removal of Cu, Fe, Ni, Zn mixtures | [106] |
Rhodococcus spp., Pseudomonas spp., | Alginate, Polyacrylamide, PVA-alginate | Removal of benzene | [107,108] |
Chlorella sorokiniana, Synechococcus spp., Spirulina platensis, | Luffa cylindrica sponge, Silica gel | Removes cadmium | [109,110,111] |
Chlorella vulgaris, Scenedesmus quadricuda and Spirulina platensis, | Not mentioned | Organophosphate pesticide malathion and the heavy metals cadmium, nickel and lead | [112] |
Komagataei bacterxylinum | Polylysine-b-polyvaline GA cryogel. Gum Arabic linked with divinyl sulfone |
Water treatment, antimicrobial activity E-coli | [113] |
Pseudomonas citronellolis, | PVA bamboo-biochar beads | Toluene and hydrocarbons | [114] |
Pseudomonas fluorescens(S3X), Microbacterium oxydans (EC 29) | Hydroxyapatite | Removes zinc and cadmium ions | [115] |
Pseudomonas rhodesiae, Bacillus subtilis, Bacillus lateroporus, Nitrosomonas europaea C-31, |
Cryogel polyethylenoxide UV | Phenol, methylphenol or cresol remediation | [116,117,118] |
Trichoderma spp., | HEMA cryogel | Cyanide removal | [119] |
Komagataei bacterxylinum | Aldehyde modified dextran | Scaffolds or mammalian cell immobilization | [120,121] |
The immobilization of microbial cells is an intriguing strategy that shows promise to provide wholesome water free from pollutants. While conventional techniques for immobilization of microbial cells exist (encapsulation, entrapment, cross-linking, physical adsorption), several immobilization technologies are also being developed and would chart the future of environmental bioremediation and protection. Some of the emerging methods include the use of 3D printed bio-coatings, enzyme aggregates, bio-reactive permeable barriers, new carriers, eco-friendly microbial consortiums, and engineered microbial strains. These may be used as stand-alone tools or integrated with nanotechnological, omics, and electrokinetic approaches, in a bid to enhance the removal of pollutants from wastewater. This would ensure the reduction of health risks posed by the use of polluted or contaminated water derived from various water ecosystems. Furthermore, new cell immobilization techniques have immense potential to yield new findings that may be exploited at all stages of wastewater treatment. However, to expand scientific insights into underlying mechanisms of action of emerging tools, a wide research gap remains to be filled. Likewise, despite the pros and cons associated with microbial cell immobilization techniques, many of the drawbacks constitute subjects for future scientific inquiry and may yet be circumvented.
The authors declare they have not used Artificial Intelligence (AI) tools in the creation of this article.
All authors (F.A.O., O.B.O., C.O.A., C.E.A., M.J.N and T.K.M) contributed to the conceptualization and writing of the original draft, as well as reviewed and carried out the final editing of this manuscript.
The authors declare no competing interests.
[1] |
Owa FD (2013) Water pollution:Sources, effects, control and management. Mediterranean J. Social Sci 4:66. https://doi.org/10.5901/mjss.2013.v4n8p65 doi: 10.5901/mjss.2013.v4n8p65
![]() |
[2] | Kumar RDH, Lee SM (2012) Water pollution and treatment technologies. J Environ Anal Toxicol 2: e103. https://doi.org/10.4172/2161-0525.1000e103 |
[3] | Ministry of Environmental Protection, MEP releases the 2014 Report on the state of environment in China, 2014. Available from: https://english.mee.gov.cn/News_service/news_release/201506/t20150612_303436.shtml. |
[4] | Miao Y, Fan C, Guo J (2012) China's water environmental problems and improvement measures. Environ Resour Econ 3:43-44. |
[5] | World Bank Group, Pakistan - Strategic country environmental assessment, Main Report no. 36946-PK World Bank, 2006. Available from: http://documents.worldbank.org/curated/en/132221468087836074/Main-report |
[6] |
Cutler DM, Miller G (2005) The role of public health improvements in health advances:The twentieth-century United States. Demography 42:1-22. https://doi.org/10.1353/dem.2005.0002 doi: 10.1353/dem.2005.0002
![]() |
[7] |
Jalan J, Ravallion M (2003) Does piped water reduce diarrhea for children in rural India? J Econ 112:153-173. https://doi.org/10.1016/S0304-4076(02)00158-6 doi: 10.1016/S0304-4076(02)00158-6
![]() |
[8] | Roushdy R, Sieverding M, Radwan H (2012) The impact of water supply and sanitation on child health: Evidence from Egypt. New York Population Council, New York, 1–72. Available from: https://doi.org/10.31899/pgy3.1016 |
[9] |
Lu YL, Song S, Wang RS, et al. (2015) Impacts of soil and water pollution on food safety and health risks in China. Environ Int 77:5-15. https://doi.org/10.1016/j.envint.2014.12.010 doi: 10.1016/j.envint.2014.12.010
![]() |
[10] | Lin NF, Tang J, Ismael HSM (2000) Study on environmental etiology of high incidence areas of liver cancer in China. World J. Gastroenterol 6:572-576. |
[11] |
Morales-Suarez-Varela MM, Llopis-Gonzalez A, Tejerizo-Perez ML (1995) Impact of nitrates in drinking water on cancer mortality in Valencia, Spain. Eur J Epidemiol 11:15-21. https://doi.org/10.1007/BF01719941 doi: 10.1007/BF01719941
![]() |
[12] |
Ebenstein A (2012) The consequences of industrialization:Evidence from water pollution and digestive cancers in China. Rev Econ Stats 94:186-201. https://doi.org/10.1162/REST_a_00150 doi: 10.1162/REST_a_00150
![]() |
[13] |
Teh CY, Budiman PM, Shak KPY, et al. (2016) Recent advancement of coagulation-flocculation and its application in wastewater treatment. Ind Eng Chem Res 55:4363-4389. https://doi.org/10.1021/acs.iecr.5b04703 doi: 10.1021/acs.iecr.5b04703
![]() |
[14] | Pontius FW (1990) Water quality and treatment. (4th Ed), New York: McGrawHill, Inc. |
[15] | Xiaofan Z, Shaohong Y, Lili M, et al. (2015) The application of immobilized microorganism technology in wastewater treatment. 2nd International Conference on Machinery, Materials Engineering, Chemical Engineering and Biotechnology (MMECEB 2015). Pp. 103–106. |
[16] | Malovanyy M, Masikevych A, Masikevych Y, et al. (2022) Use of microbiocenosis immobilized on carrier in technologies of biological treatment of surface and wastewater. J Ecol Eng 23:34-43.https: //doi.org/10.12911/22998993/151146 |
[17] |
Wang L, Cheng WC, Xue ZF, et al. (2023) Study on Cu-and Pb-contaminated loess remediation using electrokinetic technology coupled with biological permeable reactive barrier. J Environ Manage 348:119348. https://doi.org/10.1016/j.jenvman.2023.119348 doi: 10.1016/j.jenvman.2023.119348
![]() |
[18] |
Wang L, Cheng WC, Xue ZF, et al. (2024) Struvite and ethylenediaminedisuccinic acid (EDDS) enhance electrokinetic-biological permeable reactive barrier removal of copper and lead from contaminated loess. J Environ Manage 360:121100. https://doi.org/10.1016/j.jenvman.2024.121100 doi: 10.1016/j.jenvman.2024.121100
![]() |
[19] |
Dombrovskiy KO, Rylskyy OF, Gvozdyak PI (2020) The periphyton structural organization on the fibrous carrier "viya" over the waste waters purification from the oil products. Hydrobiol J 56:87-96. https://doi.org/10.1615/HydrobJ.v56.i3.70 doi: 10.1615/HydrobJ.v56.i3.70
![]() |
[20] |
Zhang Y, Piao M, He L, (2020) Immobilization of laccase on magnetically separable biochar for highly efficient removal of bisphenol A in water. RSC Adv 10:4795-4804. https://doi.org/10.1039/C9RA08800H doi: 10.1039/C9RA08800H
![]() |
[21] |
Najim AA, Radeef AY, al-Doori I, et al. (2024) Immobilization:the promising technique to protect and increase the efficiency of microorganisms to remove contaminants. J Chem Technol Biotechnol 99:1707-1733. https://doi.org/10.1002/jctb.7638 doi: 10.1002/jctb.7638
![]() |
[22] |
Zhang K, Luo X, Yang L, et al. (2021) Progress toward hydrogels in removing heavy metals from water:Problems and solutions-A review. ACS ES&T Water 1:1098-1116. https://doi.org/10.1021/acsestwater.1c00001 doi: 10.1021/acsestwater.1c00001
![]() |
[23] | Olaniran NS (1995) Environment and health: An introduction, In Olaniran NS et al. (Eds) Environment and Health. Lagos, Nigeria: Macmillan, for NCF, 34–151. |
[24] |
Singh G, Kumari B, Sinam G, (2018) Fluoride distribution and contamination in the water, soil and plants continuum and its remedial technologies, an Indian perspective-A review.Environ Poll239:95-108. https://doi.org/10.1016/j.envpol.2018.04.002 doi: 10.1016/j.envpol.2018.04.002
![]() |
[25] |
Schwarzenbach RP, Escher BI, Fenner K, (2006) The challenge of micropollutants in aquatic systems. Science 313:1072-1077. https://doi.org/10.1126/science.1127291 doi: 10.1126/science.1127291
![]() |
[26] |
Ma J, Ding Z, Wei G, et al. (2009) Sources of water pollution and evolution of water quality in the Wuwei basin of Shiyang river, Northwest China.J Environ Manage90:1168-1177. https://doi.org/10.1016/j.jenvman.2008.05.007 doi: 10.1016/j.jenvman.2008.05.007
![]() |
[27] | Abdulmumini A, Gumel SM, Jamil G (2015) Industrial effluents as major source of water pollution in Nigeria:An overview. Am J Chem Appl 1:45-50. |
[28] | Fakayode O (2005) Impact assessment of industrial effluent on water quality of the receiving Alaro River in Ibadan. Nigerian Afr J Environ Assoc Manage 10:1-13 |
[29] |
Begum A, Ramaiah M, Harikrishna, I, (2009) Heavy metals pollution and chemical profile of Cauvery of river water. J Chem 6:45-52. https://doi.org/10.1155/2009/154610 doi: 10.1155/2009/154610
![]() |
[30] | Sunita S, Darshan M, Jayita T, (2014) A comparative analysis of the physico-chemical properties and heavy metal pollution in three major rivers across India. Int J Sci Res 3:1936-1941. |
[31] |
Li J, Yang Y, Huan H, et al. (2016) Method for screening prevention and control measures and technologies based on groundwater pollution intensity assessment. Sci Total Environ 551:143-154. https://doi.org/10.1016/j.scitotenv.2015.12.152 doi: 10.1016/j.scitotenv.2015.12.152
![]() |
[32] |
Yuanan H, Hefa C (2013) Water pollution during China's industrial transition. Environ Dev 8:57-73. https://doi.org/10.1016/j.envdev.2013.06.001 doi: 10.1016/j.envdev.2013.06.001
![]() |
[33] |
Li W, Hongbin W (2021) Control of urban river water pollution is studied based on SMS. Environ Technol Innov 22:101468. https://doi.org/10.1016/j.eti.2021.101468 doi: 10.1016/j.eti.2021.101468
![]() |
[34] | Swapnil MK (2014) Water pollution and public health issues in Kolhapur city in Maharashtra. Int J Sci Res Pub 4:1-6. |
[35] |
Qijia C, Yong H, Hui W, (2019) Diversity and abundance of bacterial pathogens in urban rivers impacted by domestic sewage. Environ Pollut 249:24-35. https://doi.org/10.1016/j.envpol.2019.02.094 doi: 10.1016/j.envpol.2019.02.094
![]() |
[36] | Ministry of Environmental Protection (MEP), 2011 China State of the Environment. China Environmental Science Press, Beijing, China, 2012. |
[37] |
Gao C, Zhang T (2010) Eutrophication in a Chinese context:Understanding various physical and socio-economic aspects. Ambio 39:385-393. https://doi.org/10.1007/s13280-010-0040-5 doi: 10.1007/s13280-010-0040-5
![]() |
[38] | Chanti BP, Prasadu DK (2015) Impact of pharmaceutical wastes on human life and environment. RCJ 8:67-70. |
[39] | World Health Organization, WHO (2013) Water sanitation and health. Available from: https://www.who.int/teams/environment-climate-change-and-health/water-sanitation-and-health |
[40] | Sayadi MH, Trivedy RK, Pathak RK (2010) Pollution of pharmaceuticals in environment. J Ind Pollut Control |
[41] |
Fick J, Söderström H, Lindberg RH, (2009) Contamination of surface, ground, and drinking water from pharmaceutical production. Environ Toxicol Chem 28:2522-2527. https://doi.org/10.1897/09-073.1 doi: 10.1897/09-073.1
![]() |
[42] | Rosen M, Welander T, Lofqvist A (1998) Development of a new process for treatment of a pharmaceutical wastewater. Water Sci Technol 37:251-258. |
[43] | Niraj ST, Attar SJ, Mosleh MM (2011) Sewage/wastewater treatment technologies. Sci Revs Chem Commun 1:18-24 |
[44] |
Martins SCS, Martins CM, Oliveira, Fiúza LMCG, (2013) Immobilization of microbial cells:A promising tool for treatment of toxic pollutants in industrial wastewater. Afr J Biotechnol 12:4412-4418. https://doi.org/10.5897/AJB12.2677 doi: 10.5897/AJB12.2677
![]() |
[45] |
Cassidy MB, Lee H, Trevors JT (1996) Environmental applications of immobilized microbial cells:A review. J Ind Microbiol 16:79-101. https://doi.org/10.1007/BF01570068 doi: 10.1007/BF01570068
![]() |
[46] |
Wada M, Kato J, Chibata I (1979) A new immobilization of microbial cells. Appl Microbiol Biotechnol 8:241-247. https://doi.org/10.1007/BF00508788 doi: 10.1007/BF00508788
![]() |
[47] |
Park JK, Chang HN (2000) Microencapsulation of microbial cells. Biotechnol Adv 18:303-319. https://doi.org/10.1016/S0734-9750(00)00040-9 doi: 10.1016/S0734-9750(00)00040-9
![]() |
[48] |
Mrudula S, Shyam N (2012) Immobilization of Bacillus megaterium MTCC 2444 by Ca-alginate entrapment method for enhanced alkaline protease production. Braz Arch Biol Technol 55:135-144. https://doi.org/10.1590/S1516-89132012000100017 doi: 10.1590/S1516-89132012000100017
![]() |
[49] | Xia B, Zhao Q, Qu Y (2010) The research of different immobilized microorganisms' technologies and carriers in sewage disposal. Sci Technol Inf 1:698-699. |
[50] |
Han M, Zhang C, Ho SH (2023) Immobilized microalgal system:An achievable idea for upgrading current microalgal wastewater treatment. ESE 1:100227. https://doi.org/10.1016/j.ese.2022.100227 doi: 10.1016/j.ese.2022.100227
![]() |
[51] |
Wang Z, Ishii S, Novak PJ (2021) Encapsulating microorganisms to enhance biological nitrogen removal in wastewater:recent advancements and future opportunities. Environ Sci:Water Sci Technol 7:1402-1416. https://doi.org/10.1039/D1EW00255D doi: 10.1039/D1EW00255D
![]() |
[52] |
Tong CY, Derek CJ (2023) Bio-coatings as immobilized microalgae cultivation enhancement:A review. Sci Total Environ 20:163857. https://doi.org/10.1016/j.scitotenv.2023.163857 doi: 10.1016/j.scitotenv.2023.163857
![]() |
[53] |
Dzionek A, Wojcieszyńska D, Guzik U (2022) Use of xanthan gum for whole cell immobilization and its impact in bioremediation-a review. Bioresour Technol 351:126918. https://doi.org/10.1016/j.biortech.2022.126918 doi: 10.1016/j.biortech.2022.126918
![]() |
[54] | Blanco A, Sampedro MA, Sanz B, et al. (2023) Immobilization of non-viable cyanobacteria and their use for heavy metal adsorption from water. In Environmental biotechnology and cleaner bioprocesses, CRC Press, 2023,135–153. https://doi.org/10.1201/9781003417163-14 |
[55] |
Saini S, Tewari S, Dwivedi J, et al. (2023) Biofilm-mediated wastewater treatment:a comprehensive review. Mater Adv 4:1415-1443. https://doi.org/10.1039/D2MA00945E doi: 10.1039/D2MA00945E
![]() |
[56] |
Bouabidi ZB, El-Naas MH, Zhang Z (2019) Immobilization of microbial cells for the biotreatment of wastewater:A review. Environ Chem Lett 17:241-257. https://doi.org/10.1007/s10311-018-0795-7 doi: 10.1007/s10311-018-0795-7
![]() |
[57] |
Fortman DJ, Brutman JP, De Hoe GX, et al. (2018) Approaches to sustainable and continually recyclable cross-linked polymers. ACS Sustain Chem Eng 6:11145-11159. https://doi.org/10.1021/acssuschemeng.8b02355 doi: 10.1021/acssuschemeng.8b02355
![]() |
[58] |
Mahmoudi C, Tahraoui DN, Mahmoudi H, et al. (2024) Hydrogels based on proteins cross-linked with carbonyl derivatives of polysaccharides, with biomedical applications. Int J Mol Sci 25:7839. https://doi.org/10.3390/ijms25147839 doi: 10.3390/ijms25147839
![]() |
[59] | Waheed A, Mazumder MAJ, Al-Ahmed A, et al. (2019) Cell encapsulation. In Jafar MM, Sheardown H, Al-Ahmed A (Eds), Functional biopolymers, Polymers and polymeric composites: A reference series. Springer, Cham. https://doi.org/10.1007/978-3-319-95990-0_4 |
[60] |
Chen S, Arnold WA, Novak PJ (2021) Encapsulation technology to improve biological resource recovery:recent advancements and research opportunities. Environ Sci Water Res Technol 7:16-23. https://doi.org/10.1039/D0EW00750A doi: 10.1039/D0EW00750A
![]() |
[61] | Tripathi A, Melo JS (2021) Immobilization Strategies:Biomedical, Bioengineering and Environmental Applications (Gels Horizons:From Science to Smart Materials), 676. https://doi.org/10.1007/978-981-15-7998-1p |
[62] | Górecka E, Jastrzębska M (2011) Immobilization techniques and biopolymer carriers. Biotechnol Food Sci 75:65-86. |
[63] |
Lopez A, Lazaro N, Marques AM (1997) The interphase technique:a simple method of cell immobilization in gel-beads. J of Microbiol Methods 30:231-234. https://doi.org/10.1016/S0167-7012(97)00071-7 doi: 10.1016/S0167-7012(97)00071-7
![]() |
[64] | Ramakrishna SV, Prakasha RS (1999) Microbial fermentations with immobilized cells. Curr Sci 77:87-100. |
[65] |
Saberi RR, Skorik YA, Thakur VK, et al. (2021) Encapsulation of plant biocontrol bacteria with alginate as a main polymer material. Int J Mol Sci 22:11165. https://doi.org/10.3390/ijms222011165 doi: 10.3390/ijms222011165
![]() |
[66] |
Mohidem NA, Mohamad M, Rashid MU, et al. (2023) Recent advances in enzyme immobilisation strategies:An overview of techniques and composite carriers.J Compos Sci7:488. https://doi.org/10.3390/jcs7120488 doi: 10.3390/jcs7120488
![]() |
[67] |
Thomas D, O'Brien T, Pandit A (2018) Toward customized extracellular niche engineering:progress in cell-entrapment technologies. Adv Mater 30:1703948. https://doi.org/10.1002/adma.201703948 doi: 10.1002/adma.201703948
![]() |
[68] |
Farasat A, Sefti MV, Sadeghnejad S, et al. (2017) Mechanical entrapment analysis of enhanced preformed particle gels (PPGs) in mature reservoirs.J Pet Sci Eng157:441-450. https://doi.org/10.1016/j.petrol.2017.07.028 doi: 10.1016/j.petrol.2017.07.028
![]() |
[69] |
Sampaio CS, Angelotti JA, Fernandez-Lafuente R, et al. (2022) Lipase immobilization via cross-linked enzyme aggregates:Problems and prospects-A review. Int J Biol Macromol 215:434-449. https://doi.org/10.1016/j.ijbiomac.2022.06.139 doi: 10.1016/j.ijbiomac.2022.06.139
![]() |
[70] |
Picos-Corrales LA, Morales-Burgos AM, Ruelas-Leyva JP, et al. (2023) Chitosan as an outstanding polysaccharide improving health-commodities of humans and environmental protection.Polymers 15:526. https://doi.org/10.3390/polym15030526 doi: 10.3390/polym15030526
![]() |
[71] |
Gill J, Orsat V, Kermasha S (2018) Optimization of encapsulation of a microbial laccase enzymatic extract using selected matrices. Process Biochem 65:55-61. https://doi.org/10.1016/j.procbio.2017.11.011 doi: 10.1016/j.procbio.2017.11.011
![]() |
[72] |
Navarro JM, Durand G (1977) Modification of yeast metabolism by immobilization onto porous glass. Eur J Appl Microbiol 4:243-254. https://doi.org/10.1007/BF00931261 doi: 10.1007/BF00931261
![]() |
[73] |
Alkayyali T, Cameron T, Haltli B, et al. (2019) Microfluidic and cross-linking methods for encapsulation of living cells and bacteria-A review. Analytica Chimica Acta 1053:1-21. https://doi.org/10.1016/j.aca.2018.12.056 doi: 10.1016/j.aca.2018.12.056
![]() |
[74] |
Guisan JM, Fernandez-Lorente G, Rocha-Martin J, et al. (2022) Enzyme immobilization strategies for the design of robust and efficient biocatalysts. CRGSC 35:100593. https://doi.org/10.1016/j.cogsc.2022.100593 doi: 10.1016/j.cogsc.2022.100593
![]() |
[75] |
Qi F, Jia Y, Mu R, et al. (2021) Convergent community structure of algal bacterial consortia and its effect on advanced wastewater treatment and biomass production. Sci Rep 11:21118. https://doi.org/10.1038/s41598-021-00517-x doi: 10.1038/s41598-021-00517-x
![]() |
[76] |
Sharma M, Agarwal S, Agarwal MR, et al. (2023) Recent advances in microbial engineering approaches for wastewater treatment:a review. Bioengineered 14:2184518. https://doi.org/10.1080/21655979.2023.2184518 doi: 10.1080/21655979.2023.2184518
![]() |
[77] |
Cortez S, Nicolau A, Flickinger MC, et al. (2017) Biocoatings:A new challenge for environmental biotechnology. Biochem Eng J 121:25-37. https://doi.org/10.1016/j.bej.2017.01.004 doi: 10.1016/j.bej.2017.01.004
![]() |
[78] | Zhao LL, Pan B, Zhang W, et al. (2011) Polymer-supported nanocomposites for environmental application:a review Chem. Eng. J., 170:381-394. https://doi.org/10.1016/j.cej.2011.02.071 |
[79] |
Flickinger MC, Schottel JL, Bond DR (2007) Scriven Painting and printing living bacteria:Engineering nanoporous biocatalytic coatings to preserve microbial viability and intensify reactivity. Biotechnol Prog 23:2-17. https://doi.org/10.1021/bp060347r doi: 10.1021/bp060347r
![]() |
[80] |
Martynenko NN, Gracheva IM, Sarishvili NG, et al. (2004) Immobilization of champagne yeasts by inclusion into cryogels of polyvinyl alcohol:Means of preventing cell release from the carrier matrix. Appl Biochem Microbiol 40:158-164. https://doi.org/10.1023/B:ABIM.0000018919.13036.19 doi: 10.1023/B:ABIM.0000018919.13036.19
![]() |
[81] |
Yang D, Shu-Qian F, Yu S, et al. (2015) A novel biocarrier fabricated using 3D printing technique for wastewater treatment. Sci Rep 5:12400. https://doi.org/10.1038/srep12400 doi: 10.1038/srep12400
![]() |
[82] |
Ayilara MS, Babalola OO (2023) Bioremediation of environmental wastes:the role of microorganisms. Front Agron 5:1183691. https://doi.org/10.3389/fagro.2023.1183691 doi: 10.3389/fagro.2023.1183691
![]() |
[83] | Kumar V, Garg VK, Kumar S, et al. (2022) Omics for environmental engineering and microbiology systems (Florida:CRC Press). https://doi.org/10.1201/9781003247883 |
[84] |
Liu L, Wu Q, Miao X, et al. (2022) Study on toxicity effects of environmental pollutants based on metabolomics:A review. Chemosphere 286:131815. https://doi.org/10.1016/j.chemosphere.2021.131815 doi: 10.1016/j.chemosphere.2021.131815
![]() |
[85] |
Zdarta J, Jankowska K, Bachosz K, et al. (2021) Enhanced wastewater treatment by immobilized enzymes. Current Pollution Reports 7:167-179. https://doi.org/10.1007/s40726-021-00183-7 doi: 10.1007/s40726-021-00183-7
![]() |
[86] | Freeman A, Lilly MD (1998) Effect of processing parameters on the feasibility and operational stability of immobilized viable microbial cells. Enzyme Microb Technol 23 335-345. https://doi.org/10.1016/S0141-0229(98)00046-5 |
[87] | Ligler FS, Taitt CR (2011) Optical biosensors: Today and tomorrow, Elsevier, Pp. 151. |
[88] |
Ismail E, José D, Gonçalves V, et al. (2015) Principles, techniques, and applications of biocatalyst immobilization for industrial application. Appl Microbiol Biotechnol 99:2065-2082. https://doi.org/10.1007/s00253-015-6390-y doi: 10.1007/s00253-015-6390-y
![]() |
[89] |
Anselmo AM, Novais JM (1992) Degradation of phenol by immobilized mycelium of Fusarium flocciferum in continuous culture. Water Sci Technol 25:161-168.https://doi.org/10.2166/wst.1992.0024 doi: 10.2166/wst.1992.0024
![]() |
[90] | Liu Z, Yang H, Jia S (1992) Study on decolorization of dyeing wastewater by mixed bacterial cells immobilized in polyvinyl alcohol (PVA). China Environ Sci 13:2-6. |
[91] | Guomin C, Zhao Q, Gong J (2001) Study on nitrogen removal from wastewater in a new co-immobilized cells membrane bioreactor. Acta Sci Circumst. 21:189-193. |
[92] | Jogdand VG, Chavan PA, Ghogare PD, et al. (2012) Remediation of textile industry waste-water using immobilized Aspergillus terreus. Eur J Exp Biol 2:1550-1555 |
[93] |
Adlercreutz P, Holst O, Mattiasson B (1982) Oxygen supply to immobilized cells:2. Studies on a coimmobilized algae-bacteria preparation with in situ oxygen generation. Enzyme Microb Tech 4:395-400. https://doi.org/10.1016/0141-0229(82)90069-2 doi: 10.1016/0141-0229(82)90069-2
![]() |
[94] |
Chevalier P, de la Noüe J (1988) Behavior of algae and bacteria co-immobilized in carrageenan, in a fluidized bed. Enzyme Microb Tech 10:19-23. https://doi.org/10.1016/0141-0229(88)90093-2 doi: 10.1016/0141-0229(88)90093-2
![]() |
[95] |
Wikström P, Szwajcer E, Brodelius P, et al. (1982) Formation of α-keto acids from amino acids using immobilized bacteria and algae. Biotechnol Lett 4:153-158. https://doi.org/10.1007/BF00144316 doi: 10.1007/BF00144316
![]() |
[96] |
Serebrennikova MK, Golovina EE, Kuyukina MS, et al. (2017) A consortium of immobilized Rhodococci for oil field wastewater treatment in a column bioreactor. Appl Biochem Microbiol 53:435-440. https://doi.org/10.1134/S0003683817040123 doi: 10.1134/S0003683817040123
![]() |
[97] |
Choi M, Chaudhary R, Lee M, et al. (2020) Enhanced selective enrichment of partial nitritation and anammox bacteria in a novel two-stage continuous flow system using flat-type poly(vinylalcohol) cryogel films. Bioresour Technol 300:122546. https://doi.org/10.1016/j.biortech.2019.122546 doi: 10.1016/j.biortech.2019.122546
![]() |
[98] |
Yordanova G, Ivanova D, Godjevargova T, et al. (2009) Biodegradation of phenol by immobilized Aspergillus awamori NRRL 3112 on modified polyacrylonitrile membrane. Biodegradation 20:717-726. https://doi.org/10.1007/s10532-009-9259-x doi: 10.1007/s10532-009-9259-x
![]() |
[99] | Tekere M (2019) Microbial bioremediation and different bioreactors designs applied. In Biotechnology and Bioengineering; IntechOpen: London, UK, Pp. 1–19. https://doi.org/10.5772/intechopen.83661 |
[100] |
Liu SH, Zeng ZT, Niu QY, et al. (2019) Influence of immobilization on phenanthrene degradation by Bacillus sp. P1 in the presence of Cd (II). Sci Total Environ 655:1279-1287. https://doi.org/10.1016/j.scitotenv.2018.11.272 doi: 10.1016/j.scitotenv.2018.11.272
![]() |
[101] |
Liu SH, Lin HH, Lai CY, et al. (2019) Microbial community in a pilot-scale biotrickling filter with cell-immobilized biochar beads and its performance in treating toluene-contaminated waste gases. Int Biodeterior Biodegrad 144:104743. https://doi.org/10.1016/j.ibiod.2019.104743 doi: 10.1016/j.ibiod.2019.104743
![]() |
[102] |
Önnby L, Pakade V, Mattiasson B, et al. (2012) Polymer composite adsorbents using particles of molecularly imprinted polymers or aluminium oxide nanoparticles for treatment of arsenic contaminated waters. Water Res 46:4111-4120. https://doi.org/10.1016/j.watres.2012.05.028 doi: 10.1016/j.watres.2012.05.028
![]() |
[103] |
Baimenov A, Berillo D, Azat S, et al. (2020) Removal of Cd2+ from water by use of super-macroporous cryogels and comparison to commercial adsorbents. Polymers 12:2405. https://doi.org/10.3390/polym12102405 doi: 10.3390/polym12102405
![]() |
[104] |
Baimenov AZ, Berillo DA, Moustakas K, et al. (2020) Efficient removal of mercury (II) from water by use of cryogels and comparison to commercial adsorbents under environmentally relevant conditions. J Hazard Mater 399:123056. https://doi.org/10.1016/j.jhazmat.2020.123056 doi: 10.1016/j.jhazmat.2020.123056
![]() |
[105] |
Safonova E, Kvitko KV, Iankevitch MI, et al. (2004) Biotreatment of industrial wastewater by selected algal-bacterial consortia. Eng Life Sci 4:347-353. https://doi.org/10.1002/elsc.200420039 doi: 10.1002/elsc.200420039
![]() |
[106] |
Blanco A, Sanz B, Llama MJ, et al. (1999) Biosorption of heavy metals to immobilized Phormidium laminosum biomass. J Biotechnol 69:227-240. https://doi.org/10.1016/S0168-1656(99)00046-2 doi: 10.1016/S0168-1656(99)00046-2
![]() |
[107] |
Somerville HJ, Mason JR, Ruffell RN (1977) Benzene degradation by bacterial cells immobilized in polyacrylamide gel. Appl Microbiol Biotechnol 4:75-85. https://doi.org/10.1007/BF00929158 doi: 10.1007/BF00929158
![]() |
[108] |
Tsai SL, Lin CW, Wu CH, et al. (2013) Kinetics of xenobiotic biodegradation by the Pseudomonas sp. YATO411 strain in suspension and cell-immobilized beads. J Taiwan Inst Chem Eng 44:303-309. https://doi.org/10.1016/j.jtice.2012.11.004 doi: 10.1016/j.jtice.2012.11.004
![]() |
[109] |
Akhtar N, Saeed A, Iqbal M (2003) Chlorella sorokiniana immobilized on the biomatrix of vegetable sponge of Luffa cylindrica:a new system to remove cadmium from contaminated aqueous medium. Bioresour Technol 88:163-165. https://doi.org/10.1016/S0960-8524(02)00289-4 doi: 10.1016/S0960-8524(02)00289-4
![]() |
[110] |
Saeed A, Iqbal M (2006) Immobilization of blue green microalgae on loofa sponge to biosorb cadmium in repeated shake flask batch and continuous flow fixed bed column reactor system. World J Microb Biotechnol 22:775-782. https://doi.org/10.1007/s11274-005-9103-3 doi: 10.1007/s11274-005-9103-3
![]() |
[111] |
Rangasayatorn N, Pokethitiyook P, Upatahm ES, et al. (2004) Cadmium biosorption by cells of Spirulina platensis TISTR 8217 immobilized in alginate and silica gel. Environ Int 30:57-63. https://doi.org/10.1016/S0160-4120(03)00146-6 doi: 10.1016/S0160-4120(03)00146-6
![]() |
[112] | Mohamed AA, Ahmed MA, Mahmoud ME, et al. (2019) Bioremediation of a pesticide and selected heavy metals in wastewater from various sources using a consortium of microalgae and cyanobacteria. Slov Vet Res 56:61-74. |
[113] |
Aslıyüce S, Denizli A (2017) Design of PHEMA Cryogel as Bioreactor Matrices for Biological Cyanide Degradation from Waste-water. Hacet J Biol Chem 45:639-645. https://doi.org/10.15671/HJBC.2018.208 doi: 10.15671/HJBC.2018.208
![]() |
[114] |
Suner SS, Sahiner N (2018) Humic acid particle embedded super porous gum Arabic cryogel network for versatile use. Polym Adv Technol 29:151-159. https://doi.org/10.1002/pat.4097 doi: 10.1002/pat.4097
![]() |
[115] | Sharma A, Bhat S, Vishnoi T, et al. (2013). Three-dimensional super macroporous carrageenan-gelatin cryogel matrix for tissue engineering applications. BioMed Res Int 2013: 478279. |
[116] |
Le Noir M, Plieva FM, Mattiasson B (2009) Removal of endocrine-disrupting compounds from water using macroporous molecularly imprinted cryogels in a moving-bed reactor. J Sep Sci 32:1471-1479. https://doi.org/10.1002/jssc.200800670 doi: 10.1002/jssc.200800670
![]() |
[117] |
See S, Lim PE, Lim JW, et al. (2005) Evaluation of o-cresol removal using PVA-cryogel-immobilised biomass enhanced by PAC. Water SA 41:55-60. https://doi.org/10.4314/wsa.v41i1.8 doi: 10.4314/wsa.v41i1.8
![]() |
[118] |
Gao S, Wang Y, Diao X, et al. (2010) Effect of pore diameter and cross-linking method on the immobilization efficiency of Candida rugose lipase in SBA-15. Bioresour Technol 101:3830-3837. https://doi.org/10.1016/j.biortech.2010.01.023 doi: 10.1016/j.biortech.2010.01.023
![]() |
[119] |
Stepanov N, Efremenko E (2018) Deceived concentrated immobilized cells as biocatalyst for intensive bacterial cellulose production from various sources. Catalysts 8:33. https://doi.org/10.3390/catal8010033 doi: 10.3390/catal8010033
![]() |
[120] |
Zaushitsyna O, Berillo D, Kirsebom H, et al. (2014) Cryostructured and crosslinked viable cells forming monoliths suitable for bioreactor applications. Top Catal 57:339-348. https://doi.org/10.1007/s11244-013-0189-9 doi: 10.1007/s11244-013-0189-9
![]() |
[121] |
Qian L, Zhang H (2011) Controlled freezing and freeze drying:A versatile route for porous and micro-/nano-structured materials. J Chem Technol Biotechnol 86:172-184. https://doi.org/10.1002/jctb.2495 doi: 10.1002/jctb.2495
![]() |
1. | Emanuel Gheorghita Armanu, Simone Bertoldi, Łukasz Chrzanowski, Irina Volf, Hermann J. Heipieper, Christian Eberlein, Benefits of Immobilized Bacteria in Bioremediation of Sites Contaminated with Toxic Organic Compounds, 2025, 13, 2076-2607, 155, 10.3390/microorganisms13010155 |
S/N | Methods | Treatments |
1 | Preliminary treatment | Removing large materials/solids in wastewater, such as cloth, paper, wood, garbage, plastics, feces, metal or glass, and gravel. |
2 | Primary treatment | Physical processes, floatation and sedimentation techniques are used in removing organic and inorganic solids, oils as well as grease. |
3 | Secondary treatment | Confiscating the remaining organic matter and suspended solids through biological treatment using a variety of microorganisms. |
4 | Advanced treatment |
Physicochemical treatment, tertiary treatment, and combined biological-physical treatments. It entails the reduction of nitrogenous oxygen demand (NOD), nutrient extraction, and more toxic substances. |
Methods | Economy | Effectiveness | Durability | Secondary Pollution | References |
Entrapment | Moderate to high Cost | Effective for retaining substances within a matrix | Slightly durable and requires higher cost of maintenance | Moderate form of pollution which occurs only when matrix degrades | [50;52,53,54,55] |
Physical adsorption | • Inexpensive due to low cost of material and processes • Suitable for many pollutants • Absorbents can be regenerated and reused |
• Moderately effective • Efficiency can be reduced due to saturation of adsorbents • This method can be less effective if the pollutants involved have high specificity |
Very durable | There is minimal risk of secondary pollution | [21] [54,55,56] |
Cross-Linking or covalent Bonds | • High cost due to complex processes and expensive chemicals. • The most expensive method of immobilization. • Though this the most expensive method, but its durability justifies its cost for long term applications. |
• Highly effective for both short- and long-term applications • Most effective for tailored applications and/ or contaminants |
Most durable solution amongst these four methods of immobilization | • Risk of secondary pollution is very low due to the stability of covalent bonds • Cross-linking agents can be toxic if degraded |
[22,57,58] |
Encapsulation | • Cost of production is moderate and it can be high depending on the material and technology used for encapsulation • More expensive when compared to physical adsorption method |
• Effectiveness of this method is high • Very useful in preventing the release of contaminants. • This method can also be designed for various applications • They can also be designed for specific applications and/or contaminants |
• Materials used in this method has a longer lifespan • This reduces the long-term costs. |
• Low and can be moderate • Improper encapsulation can lead to higher risks • Leaching of polymeric gels or other encapsulation materials can pose great risk |
[21,51,56,59,60] |
Microorganism | Polymer Type | Application | Reference(s) |
Bacillus spp., Rhodococcus spp. | Polyvinyl alcohol (PVA) cryogel | Removal of ammonia | [96,97] |
Actinobacillus succinogenes, Rhizopus oryzae spp. | PVA cryogel | Succinic, fumaric, as well as lactic acid removal | [56] |
Aspergillus awamori | Polyacrylonitrile membrane | Phenol biodegradation | [98] |
Bacillus cereus | Polyacrylamide (PAAam)- N, N'-methylenebisacrylamide (BisAAm) cryogel | Degradation of crude oil | [99] |
Bacillus spp. | PVA- H3BO3-Ca- Alg beads | Removal of PAH | [100] |
Chlorella spp. | Agar beads, agarose, carrageenan, polyurethane foam and alginate | Removes ions Zn, Pb, Ni, Cu, Cd, Hg, nitrite, NH4 and uranium |
[101,102,103,104] |
Chlorella spp., Phormidium spp., Scenedesmus obliquus, Stichococcus spp., | Capron fibers (synthetic) | Removes oil spill, heavy metals, and phenols | [105] |
Phormidium laminosum | Epoxy resin as well as polysulphone | Removal of Cu, Fe, Ni, Zn mixtures | [106] |
Rhodococcus spp., Pseudomonas spp., | Alginate, Polyacrylamide, PVA-alginate | Removal of benzene | [107,108] |
Chlorella sorokiniana, Synechococcus spp., Spirulina platensis, | Luffa cylindrica sponge, Silica gel | Removes cadmium | [109,110,111] |
Chlorella vulgaris, Scenedesmus quadricuda and Spirulina platensis, | Not mentioned | Organophosphate pesticide malathion and the heavy metals cadmium, nickel and lead | [112] |
Komagataei bacterxylinum | Polylysine-b-polyvaline GA cryogel. Gum Arabic linked with divinyl sulfone |
Water treatment, antimicrobial activity E-coli | [113] |
Pseudomonas citronellolis, | PVA bamboo-biochar beads | Toluene and hydrocarbons | [114] |
Pseudomonas fluorescens(S3X), Microbacterium oxydans (EC 29) | Hydroxyapatite | Removes zinc and cadmium ions | [115] |
Pseudomonas rhodesiae, Bacillus subtilis, Bacillus lateroporus, Nitrosomonas europaea C-31, |
Cryogel polyethylenoxide UV | Phenol, methylphenol or cresol remediation | [116,117,118] |
Trichoderma spp., | HEMA cryogel | Cyanide removal | [119] |
Komagataei bacterxylinum | Aldehyde modified dextran | Scaffolds or mammalian cell immobilization | [120,121] |
S/N | Methods | Treatments |
1 | Preliminary treatment | Removing large materials/solids in wastewater, such as cloth, paper, wood, garbage, plastics, feces, metal or glass, and gravel. |
2 | Primary treatment | Physical processes, floatation and sedimentation techniques are used in removing organic and inorganic solids, oils as well as grease. |
3 | Secondary treatment | Confiscating the remaining organic matter and suspended solids through biological treatment using a variety of microorganisms. |
4 | Advanced treatment |
Physicochemical treatment, tertiary treatment, and combined biological-physical treatments. It entails the reduction of nitrogenous oxygen demand (NOD), nutrient extraction, and more toxic substances. |
Methods | Economy | Effectiveness | Durability | Secondary Pollution | References |
Entrapment | Moderate to high Cost | Effective for retaining substances within a matrix | Slightly durable and requires higher cost of maintenance | Moderate form of pollution which occurs only when matrix degrades | [50;52,53,54,55] |
Physical adsorption | • Inexpensive due to low cost of material and processes • Suitable for many pollutants • Absorbents can be regenerated and reused |
• Moderately effective • Efficiency can be reduced due to saturation of adsorbents • This method can be less effective if the pollutants involved have high specificity |
Very durable | There is minimal risk of secondary pollution | [21] [54,55,56] |
Cross-Linking or covalent Bonds | • High cost due to complex processes and expensive chemicals. • The most expensive method of immobilization. • Though this the most expensive method, but its durability justifies its cost for long term applications. |
• Highly effective for both short- and long-term applications • Most effective for tailored applications and/ or contaminants |
Most durable solution amongst these four methods of immobilization | • Risk of secondary pollution is very low due to the stability of covalent bonds • Cross-linking agents can be toxic if degraded |
[22,57,58] |
Encapsulation | • Cost of production is moderate and it can be high depending on the material and technology used for encapsulation • More expensive when compared to physical adsorption method |
• Effectiveness of this method is high • Very useful in preventing the release of contaminants. • This method can also be designed for various applications • They can also be designed for specific applications and/or contaminants |
• Materials used in this method has a longer lifespan • This reduces the long-term costs. |
• Low and can be moderate • Improper encapsulation can lead to higher risks • Leaching of polymeric gels or other encapsulation materials can pose great risk |
[21,51,56,59,60] |
Microorganism | Polymer Type | Application | Reference(s) |
Bacillus spp., Rhodococcus spp. | Polyvinyl alcohol (PVA) cryogel | Removal of ammonia | [96,97] |
Actinobacillus succinogenes, Rhizopus oryzae spp. | PVA cryogel | Succinic, fumaric, as well as lactic acid removal | [56] |
Aspergillus awamori | Polyacrylonitrile membrane | Phenol biodegradation | [98] |
Bacillus cereus | Polyacrylamide (PAAam)- N, N'-methylenebisacrylamide (BisAAm) cryogel | Degradation of crude oil | [99] |
Bacillus spp. | PVA- H3BO3-Ca- Alg beads | Removal of PAH | [100] |
Chlorella spp. | Agar beads, agarose, carrageenan, polyurethane foam and alginate | Removes ions Zn, Pb, Ni, Cu, Cd, Hg, nitrite, NH4 and uranium |
[101,102,103,104] |
Chlorella spp., Phormidium spp., Scenedesmus obliquus, Stichococcus spp., | Capron fibers (synthetic) | Removes oil spill, heavy metals, and phenols | [105] |
Phormidium laminosum | Epoxy resin as well as polysulphone | Removal of Cu, Fe, Ni, Zn mixtures | [106] |
Rhodococcus spp., Pseudomonas spp., | Alginate, Polyacrylamide, PVA-alginate | Removal of benzene | [107,108] |
Chlorella sorokiniana, Synechococcus spp., Spirulina platensis, | Luffa cylindrica sponge, Silica gel | Removes cadmium | [109,110,111] |
Chlorella vulgaris, Scenedesmus quadricuda and Spirulina platensis, | Not mentioned | Organophosphate pesticide malathion and the heavy metals cadmium, nickel and lead | [112] |
Komagataei bacterxylinum | Polylysine-b-polyvaline GA cryogel. Gum Arabic linked with divinyl sulfone |
Water treatment, antimicrobial activity E-coli | [113] |
Pseudomonas citronellolis, | PVA bamboo-biochar beads | Toluene and hydrocarbons | [114] |
Pseudomonas fluorescens(S3X), Microbacterium oxydans (EC 29) | Hydroxyapatite | Removes zinc and cadmium ions | [115] |
Pseudomonas rhodesiae, Bacillus subtilis, Bacillus lateroporus, Nitrosomonas europaea C-31, |
Cryogel polyethylenoxide UV | Phenol, methylphenol or cresol remediation | [116,117,118] |
Trichoderma spp., | HEMA cryogel | Cyanide removal | [119] |
Komagataei bacterxylinum | Aldehyde modified dextran | Scaffolds or mammalian cell immobilization | [120,121] |