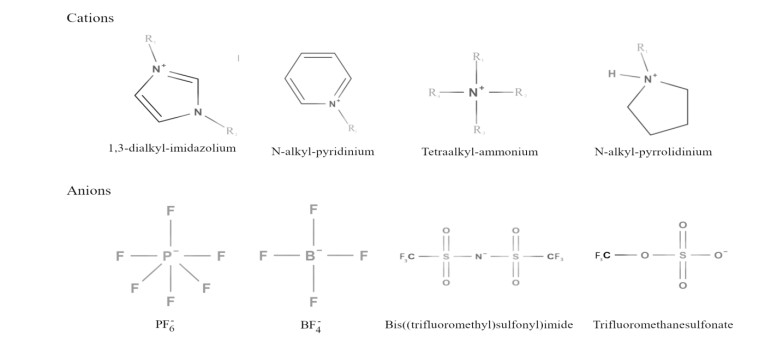
Citation: R. M. L. L. Rathnayake, K. S. Perera, K. P. Vidanapathirana. Past, present and future of ionic liquid based polymer electrolytes[J]. AIMS Energy, 2020, 8(2): 231-251. doi: 10.3934/energy.2020.2.231
[1] | W. Prasadini, Kumudu S. Perera, Kamal P. Vidanapathirana . Performance of Zn/Graphite rechargeable cells with 1-ethyl-3-methylimidazolium trifluoromethanesulfonate based gel polymer electrolyte. AIMS Energy, 2018, 6(4): 566-575. doi: 10.3934/energy.2018.4.566 |
[2] | Mohd Fareezuan Abdul Aziz, Nur Ezyanie Safie, Mohd Asyadi Azam, Tunku Aidil Ilham Tunku Adaham, Tan Jun Yu, Akito Takasaki . A comprehensive review of filler, plasticizer, and ionic liquid as an additive in GPE for DSSCs. AIMS Energy, 2022, 10(6): 1122-1145. doi: 10.3934/energy.2022053 |
[3] | W.A.D.S.S. Weerasinghe, K.P. Vidanapathirana, K.S. Perera . Performance evaluation of polyaniline-based redox capacitors with respect to polymerization current density. AIMS Energy, 2018, 6(4): 593-606. doi: 10.3934/energy.2018.4.593 |
[4] | César A. C. Sequeira, David S. P. Cardoso, Marta Martins, Luís Amaral . Novel materials for fuel cells operating on liquid fuels. AIMS Energy, 2017, 5(3): 458-481. doi: 10.3934/energy.2017.3.458 |
[5] | Christian Hellert, Christian Klemt, Uta Scheidt, Irén Juhász Junger, Eva Schwenzfeier-Hellkamp, Andrea Ehrmann . Rehydrating dye sensitized solar cells. AIMS Energy, 2017, 5(3): 397-403. doi: 10.3934/energy.2017.3.397 |
[6] | B. Onwona-Agyeman, A. Yaya, G. R. A. Kumara, M. Nakao . Light-soaking tests of zinc oxide photoanodes sensitized with an indoline dye on different transparent conductive substrates. AIMS Energy, 2018, 6(6): 949-958. doi: 10.3934/energy.2018.6.949 |
[7] | Redon Resuli, Ibrahim Turhan, Andrea Ehrmann, Tomasz Blachowicz . Textile-based batteries with nanofiber interlayer. AIMS Energy, 2018, 6(2): 261-268. doi: 10.3934/energy.2018.2.261 |
[8] | Saad S. Alrwashdeh, Falah M. Alsaraireh, Mohammad A. Saraireh, Henning Markötter, Nikolay Kardjilov, Merle Klages, Joachim Scholta, Ingo Manke . In-situ investigation of water distribution in polymer electrolyte membrane fuel cells using high-resolution neutron tomography with 6.5 µm pixel size. AIMS Energy, 2018, 6(4): 607-614. doi: 10.3934/energy.2018.4.607 |
[9] | Lawrence Moura, Mario González, Jéssica Silva, Lara Silva, Izaac Braga, Paula Ferreira, Priscila Sampaio . Evaluation of technological development of hydrogen fuel cells based on patent analysis. AIMS Energy, 2024, 12(1): 190-213. doi: 10.3934/energy.2024009 |
[10] | Gustavo Aguilar, Pranjali D. Muley, Charles Henkel, Dorin Boldor . Effects of biomass particle size on yield and composition of pyrolysis bio-oil derived from Chinese tallow tree (Triadica Sebifera L.) and energy cane (Saccharum complex) in an inductively heated reactor. AIMS Energy, 2015, 3(4): 838-850. doi: 10.3934/energy.2015.4.838 |
Fossil fuel was a major contributor to the industrial revolution and it was a reliable energy source at that period of time. However, with the use of over two centuries and the massive increase of energy demand, it is no longer a well-grounded energy source due to the depletion and the environmental concerns. Such atmosphere has lead humanity to rely on external energy sources more and more. Although mankind is majorly dependent on combustive sources, they are in a technological advantage of almost completely replacing to the electronic devices. There are various types of energy storage devices presently in the market and are continuing with the portable consumer grade appliances. However, large scale energy storages are also a demanding field today to overcome some holdbacks of renewable energy sources.
Electrochemical energy storage (EES) technologies are currently playing dominant roles in the global effort to tackle the challenges of renewable energy supply. These EES devices can be divided into few groups based on the charging discharging mechanisms such as (1) rechargeable batteries including redox flow batteries (2) super capacitors (electrochemical capacitors) and (3) various hybrids of battery and supercapacitor (supercapattery and supercabattery) [1]. Rechargeable batteries are recognized for their high energy capacity whilst supercapacitors are perceived to have high power capability and long cycle life measured against the common ground. Hybrids are proposed and made to harvest the advantages of both and reduce some of downsides as well. All these devices consist of electrodes and electrolytes which play the major roles in capacitive action coupled with ion, electron transfer [2].
Electrolytes, in liquid phase, are indispensable parts in all types of EES devices. They have higher ionic conductivity compared to other types of electrolytes and keep an electronic insulation between positive and negative electrodes. Generally, aqueous electrolytes have high ionic conductivity and operational safety, but the maximum charging voltage (MCV) of an aqueous cell is limited by the splitting voltage of water [3]. Since the energy capacity is dramatically decreased by a slight drop of MCV, alternating systems like organic electrolytes are more favourable although they are volatile and flammable, bare high environmental impact, bring safety issues and have relatively low ionic conductivity than aqueous systems in addition to the high cost and maintenance difficulty. One approach to solve some of these problems is using ionic liquid (IL) as the electrolyte material.
ILs are pure liquid salts. They are expressly highlighted by their zero or negligible volatility, highly ionized nature, broad temperature ranges of liquidity, and wide operating voltage windows. Some extra features such as low vapor pressure above 100 ℃ and good retention are available to give a value addition to ILs [4].
Since most of the ILs are liquids in room temperature, it can be used directly as an electrolyte without concerning a solvent medium. But using organic liquid electrolytes brings up a set of adverse complications to devices, such as leakage, corrosion, shortage etc.
To address these problems, scientific community later on came up with an idea to use ILs with PEs to keep the mechanical properties alongside with good electrochemical properties of ILs [5]. It is because suffering of many PEs with low ambient temperature conductivities.
Development in the field of synthetic polymer materials was rapid in the industrialized world due to its fascinating properties. Concurrently, physicochemical and theoretical tools necessary to investigate the polymeric materials were developed by various research groups. Poly (ethylene) oxide (PEO) complexes with sodium thiocyanate (NaSCN), potassium thiocyanate (KSCN) and sodium iodide were observed to have ionic conductivity by Wright et al. [6]. At that time, new discoveries in the field of macromolecular synthesis were probably not cost effective, at least in terms of the polymer industry for consumer applications. The focus shifted towards more novel fields of research such as composite materials and high-performance fibers (e.g., Kevlar) instead of researching on low performing electrolytes. In solid-state physics and chemistry, new breakthroughs were needed to rationalize the operation of electro active and optically active polymers. In 1978 Armand et al. considered the relevance of Wright’s publication and proposed to use salt-polymer complex as a solid electrolyte [6,7,8]. With rapid testing of various salt compositions, he assumed the criterion for adduct formation was dependent upon charge delocalization in the anion, and later in 1982 it was proved to be accurate with the link to the lattice energy of the corresponding salts by Papke et al. [9]. When the correlation between the ion conductivity and the amorphous phase was highlighted, Armand et al. first proposed the use of these systems as solid PEs for batteries [8]. This idea opened up a new field of research and initiated an enormous amount of research activities worldwide. Several groups independently explored the correlation between the structure and morphology of these materials with conductivity and reached the same conclusions about the connection between the amorphous phase and the ion conductivity [10,11].
In the second decade of the newborn field of PEs, a strong demand arose for very light, high performing and cheap secondary batteries with the development and the widespread diffusion of micro and portable-electronics. Thus, the interest in electrolytes became crucial. As the beginning, several strategies were developed to inhibit the crystallinity of the polymer materials because it had been observed that crystalline phases hindered ionic conductivity [12,13]. New materials and new theories were developed, including: Ratner’s model of dynamic percolation, Angell and Torrel’s decoupling index, the Williams-Landel-Ferry (WLF) equation studied by Cheredame and Watanabe [14,15,16,17]. The relations between the transport numbers and the agammaegation state of the salts in PEs were investigated in Aberdeen and St. Andrews [18,19]. In 1987, Chatani and Okamura et al. published the first full crystallographic determination of a PEO/salt complex [20].
The third decade, roughly corresponding to the 1990s was the period that saw the widespread market diffusion of lithium-ion batteries and a rapid increase in low-cost production of portable electronics. PEs based on amorphous PEO were considered as ‘classics’ [6]. The novel systems were ‘salt-rich’ or Angell’s ‘polymer-in-salt’ electrolytes and gel electrolytes that included solvent molecules in the polymer matrix [21,22,23]. However, solvent-free PEs remained important for both fundamental research and applications. Single-ion conduction systems for alkali metal batteries also attracted particular interest.
Present day world is undergoing dreadful challenges with environmental pollution due to various human activities including power generation. Hence a significant attention is being focused on sharpening devices using nontoxic materials while assuring excellent performance. In par with this, PEs are also undergoing substantial modifications such as employing natural polymers and viable substitutes for solvents and plasticizers. For the latter, ILs have been identified as a suitable clan of alternative substances [24].
Innovations in the research on PEs during the fourth decade consists of the preparation and study of hybrid inorganic–organic PEs. These materials are very promising for application in high-performance lithium secondary batteries due to their mechanical, thermal, chemical and electrochemical stability and high conductivity at room temperature [25]. Significant attention has been devoted to understanding the role of host polymer crystallinity on the ion-conducting mechanism, resulting in several competing interpretations. In addition, whole new families of materials have been devised by introducing inorganic species into the chemical composition or by developing new classes of hybrid gels and polyelectrolytes based on ILs [26,27,28]. The growth of this increasingly diverse range of innovative materials was prompted by the necessity to develop devices for the conversion and storing energy like secondary batteries, fuel cells, supercapacitors and dye sensitized solar cells (DSSC). Therefore, PEs represent one of the cornerstones of development in the area of materials for conversion and storage of energy which caused important scientific and technological outbreaks in the century.
In parallel with the growing demand for clean, reliable and globally affordable power and energy, there is a severe quest for energy technologies. This has initiated many endeavors to seek new materials and suitable design technologies. With the deepening of research activities in multi variant arenas, PEs have undergone substantial amount of progress towards high safety and appreciable efficiency. In the recent past, ionic liquids (ILs) have received a tremendous attraction as a suitable material to be incorporated with PEs due to their excellent conductivity, chemical stability, less toxicity, desired electrochemical properties etc. [29]. They are considered as room temperature molten salts having bulky asymmetric organic cations and inorgamic anions. ILs have been treated as a suitable substitute for solvents in PEs which are inherently toxic. Some have defined ILs as green solvents as they are non volatile, soluable and disable for evaporation [30]. In addition, ILs have been employed to raise the ambient temperature conductivity of PEs. Passerini et al. have reported use of the IL, N-alkyl-N-methylpyrrolidinium per fluorosulfonylimide to assist conductivity enhancement in a PE [31]. 1-ethyl-3-methylimidazolium bis(trifluoromethanesulfonyl)imide (EMIMTFSI) has been successfully used for a zinc ion conducting PE and development of amorphous phase has been evidenced clearly [32].
Depending on the nature of cation, ILs can be divided into protic and aprotic groups since their liquid character is determined by the judicious choice of IL imidazolium, pyridinium, alkylammonium, alkylphosphonium, pyrrolidinium, guanidinium etc. They can combine with inorganic anions such as halides (Cl-, Br-, I-), polyatomic inorganics (PF6-, BF4-) and polyoxometallates or organic anions such as nitrate (NO3-), trifluoromethylsulfonylimide (TFSI-), and trifluoromethanesulfonate (Tf-) to form a huge number of ILs. Few common ions used in formation of ILs are shown in Figure 1. The chemical and the physical properties (Conductivity, hydrophobicity, melting point, viscosity, solubility, etc.) of the ILs are highly dependent on these combinations as well as the substitutive groups in the cation [33]. In the case of electrolytes, conductivity plays a major role so that the balance between the interactions of ion pairs has a significant impact in the real world applications.
Attention to develop IL based PEs is very high due to their significantly good properties when comparing to the other electrolytes. Having good thermal properties while maintaining a good ionic conductivity adds up to the non-volatile, high ion density and the non-flammable nature of the system. But most of ILs are liquids in room temperature. So, it is being expected that the insertion of ILs into highly conductive polymers may increase the properties of both substances. Two common ways of insertion of ILs are (Ⅰ) polymer doping with ILs, (Ⅱ) polyaddition reaction of monomers/macro-monomers in ILs and synthesis of polymeric ILs.
Polymer doping is a simple and easy to use method that has been developing since the beginning of the polymer electrolyte concept. Casting IL/polymer mix and impregnation of ILs into pre-casted membrane are the main ways of this technique.
In solvent casting technique, first the polymer is synthesized (conventional polymers can be taken) and blended with IL to form a homogenous solution and caste on to a desired substrate. The process is assisted by a solvent which is later evaporated. Polymer materials employed include homopolymers, copolymers, hydrocarbon polymers and block copolymers. Ionic conductivity of an IL doped polymer is greatly dependent on the IL concentration of the system. The main advantage of this method is the ability to change the IL amount with respect to the polymer so that the optimum conductivity can be obtained. Although the conductivity increases with the IL amount, the critical dependent on the IL/polymeric matrix ratio is the main limiting factors of the system [34].
In the method of impregnation, first the polymer membrane is prepared and subsequently soaked in a solution of IL to synthesize the electrolyte. The polymer layer can be prepared as a porous membrane or non-porous membrane as desired [35]. Preparing a non-porous method is a straight forward process. Since higher IL concentration directly exhibits an impact on the conductivity, the higher absorption ratio is more favoured in the field. Therefore, some researchers use few advanced methods to get porous polymer structures to enhance absorption.
Above procedures mainly include polymer dissolution, solution casting and solvent evaporation. Though they are easy to operate, a large amount of solvents are consumed to dissolve the polymer which makes this process tedious and environmentally non-benign. Therefore, few alternative ways are developed to synthesize IL-GPEs later on as in the Figure 4.
The first report on preparation of ion gels by in situ radical polymerization is of a vinyl monomer [2-60 hydroxyethyl methacrylate (HEMA)] in an IL, 1-butylpyridinium tetrafluoroborate (BP-BF4) done by watanabe et al. [36]. In this process, monomer and initiator are dissolved in a real liquid electrolyte and subsequently the solution is placed on a substrate to form a gel membrane after a thermal, a UV or an electron beam initiation as shown in Figure 5 [37]. Usually the solution is placed in between the electrodes to form the gel so that the compatibility with electrodes is higher. Generally these PEs show high ionic conductivity and good interfacial contacts. Also, they are having less impurities compared to solvent casting methods. However, in situ polymerization between confined electrodes sometimes lacks reproducibility due to surface side effects and quenching effects. In this approach, a key factor is the compatibility between the polymer matrix and the IL.
Polymeric ILS (PILs) can also be synthesized using two general approaches as I) Direct polymerization of a polymerizable IL-based monomer (e.g., methacryloyl-based IL, N-5 vinylimidazolium-based IL and styrenic IL Ⅱ) Direct modification of cations of currently existing polymers such as polyvinylimidazole (PVI) and poly-4-chloromethylstyrene (PCMSt) to give the same chemical structure as PILs prepared by polymerization of IL monomers. Depending on the targeted PIL, both approaches present some advantages and disadvantages [38].
Identification of chemical structure is important since it can be used to determine whether the expected structure was obtained and to understand the dependence of the properties on the structure. The common techniques include X-ray diffraction (XRD), Fourier transform infrared spectroscopy (FT-IR), Raman spectroscopy, Mass spectrometry, Nuclear magnetic resonance spectroscopy (NMR) etc.
XRD is a non-destructive, versatile analytical technique which is used to determine the crystallinity of a prepared polymer and to identify the amorphous phase in a polymer membrane [39]. These factors are the key components of the ionic conductivity and mechanical strength of the PE.
The types of chemical bonds and functional groups in a polymer can be ascertained by FT-IR and Raman spectroscopy [40]. NMR spectroscopy including 1H NMR, 13C NMR, 7B NMR and 31P NMR become the pre-eminent technique for determining the structures of organic macromolecules. The intramolecular magnetic field around an atom in a molecule changes the resonance frequency, thus giving access to details of the electronic structure of a molecule and its individual functional groups. NMR can provide detailed information about the structure, dynamics, reaction state and chemical environment of molecules.
The morphology of surface and cross section of the polymer membrane decides directly the performance of PE. By observing the morphology of the surface and cross section with Scanning electron microscopy (SEM), Field emission scanning electron microscopy (FESEM) or Transmission electron microscopy (TEM), non-porous or porous polymer structures can be confirmed. Ma et al have studied the morphology of an IL based PE and have revealed many features that are useful for describing the properties of the respective PE [29]. Furthermore, the shapes, structures and sizes of the pores in the membranes can also be observed [41,42].
Ionic conductivity and resistance of IL based PEs as well as capacity of cells or super capacitors are influenced by the thickness of the PE layer. Generally, the thickness of a PE should not exceed 30 μm.
The porosity or the void fraction is a measure of the void space of a material. For PEs, it is defined by the ratio of void volume to apparent geometric volume of polymer membrane.
Porosity=VolumeofvoidsTotalvolume100% | (1) |
It influences directly to the uptake ability of organic liquid electrolytes and mechanical properties of the PE [43].
Electrolyte uptake refers to the ratio of the weight of the absorbed liquid electrolyte by a dry polymer membrane when it is completely swollen to a dry polymer membrane. Generally, the greater the uptake of liquid electrolyte is, the higher the ionic conductivity of a GPE can be. To determine the electrolyte uptake, a dry polymer membrane is weighed, immersed into a liquid electrolyte and then weighed again. The amount of electrolyte uptake (η) is calculated by using the equation:
η=(Wt−W0)W0∗100% | (2) |
where, W0 and Wt are the weights of the polymer membranes before and after absorbing the organic liquid electrolyte respectively [44].
The heat will cause melt or decomposition of an IL based PE if it has a poor thermal stability. Since, the energy storage devices produce heat when they are in operation, it is a necessity to test the electrolyte for its thermal stability before applying. Thermogravimetry(TG) and Differential scanning calorimetry(DSC) are common techniques to evaluate the thermal stability of PEs. TG is used to analyze the thermal stability of a polymer membrane while DSC is used to determine the phase transition of the polymer when heated [44].
There may be shrinkage of an IL based PE when it is heated which might result in a short circuit between anode and cathode. The thermal shrinkage behavior is evaluated by analyzing the thermal shrinkage ratio of a polymer membrane after it is heated at a specified temperature for > 1 h. Thermal shrinkage ratio (TSR) is calculated by using equation:
TSR=(S0−S)S0∗100% | (3) |
where, S0 is the initial surface area of the electrolyte, S is the surface area after the membrane heated and kept in the temperature for an extended period of time [44].
PEs act as conductive mediums as well as separators even with the presence of ILs. Therefore, having good mechanical properties in polymer membranes in dry and wet state is important since it has to withstand assembling process as well as the mechanical abuses in the practical usage. Tensile testing is a commonly used measuring technique for this kind of characterization.
Electrochemical stability of electrolytes decides the safe potential window for charge and discharge of a system [45]. Linear sweep voltammetry (LSV) measurements are often used to determine the electrochemical stability of electrolytes. A fixed range of potential window is applied in steps to the PE and with the increase of the potential, the current through the system is measured. The plot can be used to determine the stable potential window of electrolyte [46].
Cyclic voltammetric (CV) experiments are performed with a stationary working electrode and for the other electrode, starting from an initial potential, a linear potential sweep (potential ramp) is applied. After reaching a switching potential, the sweep is reversed and the potential returns linearly to its initial value. Although technically possible to generate higher sweep rates, in general the rate lies between 5 mv/s and 1 V/s.
It has been found that incorporation of ILs widens the electrochemical stability window.
Ionic conductivity, one of the most important properties of an electrolyte, is a measure of the ability of a substance to conduct electricity. It is closely related to the degree of crystallinity, porosity and uptake ability of the IL doped polymer membrane. Commonly, ionic conductivity of an electrolyte is characterized by Electrochemical impedance spectroscopy (EIS). The electrolyte is sandwiched between two stainless steel (blocking) electrodes and impedance measurements are taken. The ionic conductivity can be calculated by the equation given below.
σ=lRbA | (4) |
where, σ is the ionic conductivity, l is the thickness of the PE film, Rb is the bulk resistance, and A is the effective area of the PE film in contact with stainless steel electrode [47].
Ion transport mechanism in IL based PEs are similar to conventional PEs. In PEs, there are two main ion transport mechanisms available.
ⅰ. via hopping mechanism in between ion coordination sites.
ⅱ. via formation of free volume due to segmental motion of polymer backbone.
The first one is described by Arrhenius behavior where as the second one is by Vogel-Tammann-Fulcher (VTF) behaviour.
Arrhenius type relationship can be expressed by the following equation.
σ=σ0exp(−EakBT) | (5) |
where, kB is Boltzmann constant, σ is the ionic conductivity, T is the absolute temperature. Ea, the activation energy [48]. When the temperature is increased, ions in the electrolyte gain thermal energy. When they become thermally agitated, they are hopping between coordination sites. This causes the conductivity to vary according to the Arrhenius behavior [49]. Arrhenius behavior is represented by a linear plot drawn between ln (conductivity) and 1/T.
The VTF behavior can be described by
σ=AT−12exp(−EaT−T0) | (6) |
where, A is the pre-exponential factor which is associated with the number of charge carriers, Ea is activation energy which can be computed from the linear best-fit of the data from log σ versus 1/T plots. T0 stands for ideal glass transition temperature [49]. Non linear Arrhenius shape evidences the VTF behavior. Formation of free volume is assisted by the polymer segmental motion which increases with temperature. Thus, increase of temperature is responsible for ion conduction in electrolytes in this instance.
Obviously, adding ILs to a PE will increase the ion concentration. So, PEs incorporated with ILs follow either Arrhenius behavior or VTF behavior [50].
Ionic transference number, ti is a measurement of the mobility of ions. Therefore, it is essential to determine ti in order to confirm the ion mobility. One of the commonly used method for determining ti is the steady-state current method or the so called Wagner Polarization method illustrated by Choudhury and Patterson et al. [51].
In addition to Wagner Polarization method, some use DC polarization test to calculate ti. In DC polarization test, a DC voltage is applied across the PE placed between two stainless steel blocking electrodes and the corresponding current will be recorded as a function of time. The total ionic transference number (ttot) of PE is calculated by:
ttot=iT−iriT | (7) |
where 𝑖𝑇 and 𝑖r are total and residual currents respectively.
The cationic transference number (𝑡+) is measured by a combined AC/DC technique called Bruce-Vincent method. The PE with non-blocking electrodes is subjected to polarization by applying a DC voltage and resultant current will be recorded. The cell resistances will also be measured before and after polarization using impedance spectroscopy. The t+ is calculated by Bruce-Vincent’s equation:
t+=Is(V−IiRi)Ii(V−IsRs) | (8) |
where, V is the applied voltage to the cell. Ii and Is are the initial and stable-state currents, respectively. Ri and Rs are the interfacial resistances before and after the polarization respectively [48].
Few basic approaches of optimization such as changing the concentrations of components in PEs including IL and/or polymer etc, adding inorganic nanoparticles, blending are being practiced in the PE field to add extra encouragement to obtain better properties.
IL based PEs are emerged as a promising group of electrolytes with more fascinating features than conventional PEs. In general, ILs are in liquid state. By keeping a considerable amount of liquid nature in polymer matrixes, they escalate ionic conductivity of PEs at ambient temperatures. However, even with the presence of the lowest possible amount of IL, mechanical properties of PEs cannot meet the practical application due to leaking. In order to eliminate the contradiction that ionic conductivity increases with the decline in mechanical strength, the polymer material reinforcement theory is introduced into PEs. That is adding inorganic nanoparticles into the PE to improve the ionic conductivity and mechanical strength [52]. This idea was firstly introduced in 1982 when Weston et al. prepared an inorganic composite PE by doping inorganic ceramic particles into the PEO-based solid-state PE [53]. With the development of nanotechnology, many different nanoparticles such as SiO2, ZrO2, MgO, Al2O3, Nb2O5, TiO2 and so on, have been introduced into PE preparation to enhance both the ionic conductivity and the mechanical strength [54,55]. Li et al. made a series of such nanocomposite polymeric membranes (NCPMs) based on PVdF-HFP incorporated with various amounts of TiO2 nanoparticles via in situ hydrolysis of Ti(OC4H9)4 [56]. The SEM micrographs reveal that porous structures exist in the NCPMs. The morphology of the porous structures in the NCPMs changes with the incorporated amount of TiO2. In addition to providing better mechanical stability, it has been disclosed that nano fillers improve electrolyte/electrode interface as well [57]. No well accepted evidence or justification is existing to show case any interaction between ILs and nano particles. While ILs uplift conductivity by providing more ions, nano particles mainly eliminate crystalline nature and result in higher conductivities. Nonetheless, latter assures good mechanical stability. Incorporation of inorganic nanoparticles related research work is still in progress.
Blending is a simple physical manipulation with more than one kind of polymer blended evenly together in a solvent to create a new material. The performance of each polymer is retained in the given blending polymer [58]. Blend-based PEs have been widely researched in the past few decades. PVDF, PVdF-HFP, polyethylene glycol(PEG), Poly(urethane acrylate)(PUA), polyacrylonitrile(PAN) and PEO have been widely used as blend-based polymer matrices for the preparation of PEs [59,60,61]. Cross-linking which is one blending method has been used to induce dimensional stability and dynamic storage modulus of polymers. Researchers often use this technique to modify the performance of different PEs [62]. A novel solid PE based on modified LiClO4 and poly (ether-urethane) network polymer from cross-linking reaction of PEG with -(CH2CH2O)-unit was reported in 2003 by Wang et al.[62]. After gelled with LiClO4-PC solution, the ionic conductivity can be in the order of 1 mS cm−1 at room temperature.
When more than one type of monomer unit is polymerized together, the product is called a copolymer and the process is called copolymerization. Copolymers can be classified based on how these monomer units are arranged along the polymer chain [63]. PVdF-HFP is a well known copolymer due to its excellent chemical stability. It is prepared by co-polymerization of crystalline VDF and amorphous HFP units [64]. With non-solvent induced phase separation (NISPS) process, a lotus root-like porous PE based on PVdF-HFP copolymer and TiO2 nanoparticles has been prepared, whose ionic conductivity is 1.21 mS cm−1 at room temperature [65].
However, blending has been accepted as a very suitable optimization method specially for IL based PEs. It is because incorporation of IL to a PE enhances liquid nature which margins the direct application in devices due to portability as well as leakage [32]. Upon blending, mechanical integrity is reserved and hence, associating problems are minimized.
Table 1 summarizes properties, merits and demerits of PE modified by some methods.
Method | Properties | Merits | Demerits |
Changing component concentration | Mechanical properties may change; Liquid nature may arise | Thickness can be reduced | Conductivity may reduce; Difficulty in handling |
Adding inorganic nano particles | Thick film | Reduction of reactivity Increase mechanical strength | Cost may be high |
Blending | Combined properties | Many desirable properties are present simultaneously | Needs correct methods for blending |
Majority of commercially available batteries are Lithium ion batteries. They typically use electrolytes which are volatile and flammable; thus safety concerns severely limit their usefulness. Lithium ion polymer cells were proposed in early 1980s as a potential solution for safety issues [8]. But the low ambient temperature ionic conductivity forced the community to look toward (1) incorporating liquid plasticizers such as ethylene carbonate (EC), propylene carbonate (PC) or low molecular weight polymers and their derivatives (2) employing lithium salts with large and flexible anions such as lithium bistrifluoromethanesulfonyl (LiTFSI) (3) mixing high conducting inorganic nanofillers likewise. However, even these attempts were somewhat successful, the main concern of the system was still present as the flammability exists in the liquid organic solvents. In general, non-toxic, environmental friendly nature of ILs adds more value to the rechargeable battery industry. In recent years many reports have shown that incorporating ILs will provide a good outcome in the near future [66].
Supercapacitors are electrochemical devices able to store charge by exploiting the reversible adsorption of ions carried by the electrolyte onto electrode which are characterized by high electrochemical stability and wide specific surface area [67]. For their high specific power supercapacitors are playing a major role in transportation where they can be coupled with lithium batteries to provide power peaks during acceleration as well as for energy recovery during braking of vehicle. Typical operating temperature for such application is higher than the average. Therefore using ILs is one of the best strategy because of their high thermal stability, wide electrochemical stability window, good conductivity and low vapor pressure [68].
Due to their low cost, high conversion efficiency and easy fabrication, dye-sensitized solar cells (DSSCs) with IL based hole conducting elements have attracted a lot of interest [55,69]. Large number of attempts can be observed in order to improve the light harvesting and conversion efficiency such as using nano structures [70]. But problems like leakage, dye desorption, electrode corrosion caused by the use of a liquid based electrolyte affects the long term stability of the system which is a necessity of prime importance in the practical applications [71]. One of the obvious and easy substitutes for such problems is using a polymer which still keeps the most of above advantages in addition to the good long term stability. But the main drawback of them is the low conductivity of the most conventional polymers(Polyethelene oxide, PEO) in the room temperature. As many research reports suggest, using an IL as a dopant can give a significant enhancement to the conductivity in room temperature [71,72].
Proton exchange membrane fuel cells (PEMFCs) are designed with a proton exchange membrane (PEM) to conduct protons while acting as an electronic insulator and reactant barrier. The basic principle in producing electrical energy in a PEMFC is converting chemical energy liberated during the electrochemical reaction of hydrogen and oxygen. An avalanche of hydrogen is supplied to the anode where it splits into protons (hydrogen ions) and electrons. Protons travel via the PEM while electrons traverse the external route. Hence, hydrogen is serving as the fuel for the PEMFC and the charge carrier is the hydrogen ion. From cathode side, a flow of oxygen is supplied. They react with protons and electrons giving rise to production of water. Due to some of their attractive features such as low operating temperature and light weight over solid oxide fuel cells, they have been popular foe numerous applications. To overcome some demerits of PEMFCs, it has been proposed some methodologies. One attempt is employing new anhydrous proton conductors such as protic organic ionic plastic crystals (POIPCs) and protic ionic liquids, are actively studied for the development of suitable PEMs [73,74].
Natural macromolecules like cellulose, chitin, starch etc. are the earth-abundant biopolymer materials with outstanding properties such as biocompatibility and biodegradability, desired chemical stability, environmental benignancy, good mechanical strength and superior thermo stability [52,55,75,76]. Still the main problem with these substances is lack of ionic conductivity. One of the best approaches to obtain the conductivity is doping an IL into the PEs or going for Poly addition reactions. Introducing ILs will still keep most of those advantages all together with good electrochemical stability. Although using natural macromolecule based PEs are cheaper than commercially available polymers, facts such as purity, chemical composition and the compatibility between the polymers and ILs should also be considered while moving through the research process. Therefore developing natural polymer and IL based PEs is a wide open field today [77].
Introducing IL based PEs into a system may not only escalate the charge carrier concentration but also their mobility leading to a higher transference number [32]. This can upsurge the performance of commercially available batteries. ILs are assumed to be having a capability to avoid some side reactions between electrolyte and electrodes. With that, cycle number of a device with IL based PEs becomes higher improving the overall productivity. Thermal stability of ILs is also a considering factor since the devices are heated through the process of charging and discharging. Low vapor pressure of the ILs also helps the systems to maintain long term durability than the organic solvents currently using in many devices in the market [78].
The superior features of nonwovens such as low price, high porosity, good wettability to liquid electrolytes, simple manufacturing process, strong mechanical strength and so on confer them potential attractions to energy storage devices. However, the commercial nonwovens are too thick and cannot be applied in devices. Electrospinning technique may play a key role to lower the thickness of nonwovens [79].
ILs are an important class of materials due to their sufficient ionic transport abilities, good electrochemical stability and non-flammable/nonvolatile nature. These properties have the potential to improve the safety and efficiency of present commercial batteries greatly. But the high cost of ILs is the greatest barrier to overcome before going into the commercialization [80]. With the development of materials science, more and more new materials will be applied in PEs for energy storage devices. The materials with low cost, high mechanical property and high absorption of liquid electrolyte (having good compatibility and suitable structure) will win out. As a good source of liquid electrolytes, ILs gained a significant interest in the researchers worldwide [81]. In the years to come, new low cost ILs will enter the world to combine with PEs.
Polymeric ionic liquids (PILs) contain both ionic liquid-like moieties and polymer frameworks and they are emerging as alternative electrolyte/binder candidates. One of the demerits of PILs is having poor electrochemical properties due to solid nature. Although the matrix is composed of the ILs, it is harder to match the physical advantages of the liquid or gel polymer electrolyte compared to the flexibility of producing other polymer electrolytes as desired. Various research groups have been geared with attractive properties of PILs and hence new PILs with improved properties are being explored [82,83].
Hosting nano materials to IL based PEs has the potential to increase the performances of the devices significantly similar to solid PEs and other types of electrolytes [84]. Applications such as using nano porous sheets to enhance the insertion of ions are also popular in the polymer community nowadays [85].
IL-PEs are getting a rising attention for their good ionic conductivity at room temperature, wide electrochemical window, good thermal and chemical stability, good compatibility during cycling and the good electrochemical stability. Specially, with the current thirst of demand for the energy and the rapid depletion of fossil fuel, renewable energy sources are more favorable in the modern world with an address for energy storage devices. IL-PEs have potential to act as electrolytes for such energy storage devices as well as a part of energy capturing devices such as DSSCs.
Their key advantages in terms of safety in the consumer grade appliances and large scale energy storage devices are non- toxicity, inflammability and environmental friendly nature. In electric automobile industry, IL-PEs have major superiority when comparing to the electrolytes currently using in LIBs due to this added safety. However, high cost and relatively poor mechanical strength are still key barriers for the application of IL-PEs. Exploring new inexpensive polymers, synthesizing compatible ILs and developing advanced production methods to find reliable and highly effective electrolytes is the main challenge for scientists at present.
Authors wish to acknowledge National Science Foundation (RG/2017/BS/02) and Wayamba University of Sri Lanka.
No conflict of interest
[1] | Liu Z, Li G, Cui T, et al. (2017) A battery super capacitor hybrid device composed of metallic zinc, a biodegradable ionic liquid electrolyte and graphite. J Solid State Electrochem 22: 91-101. |
[2] |
Singh R, Bhattacharya B, Gupta M, et al. (2017) Electrical and structural properties of ionic liquid doped polymer gel electrolyte for dual energy storage. Int J Hydrogen Energy 42: 14602-14607. doi: 10.1016/j.ijhydene.2017.04.126
![]() |
[3] |
Yan J, Wang J, Liu H, et al. (2012) Rechargeable hybrid aqueous batteries. J Power Sources 216: 222-226. doi: 10.1016/j.jpowsour.2012.05.063
![]() |
[4] | Wang A, Xu H, Zhou Q, et al. (2017) Electrochemical performances of a new solid composite polymer electrolyte based on hyperbranched star polymer and ionic liquid for lithium ion batteries. J Solid State Electrochem 10: 2355-2364. |
[5] |
Kim GT, Appetecchi GB, Alessandrini F, et al. (2007) Solvent-free, PYR1ATFSI ionic liquid-based ternary polymer electrolyte systems: I. Electrochemical characterization. J Power Sources 171: 861-869. doi: 10.1016/j.jpowsour.2007.07.020
![]() |
[6] | Fenton DE, Parker JM, Wright PV (1973) Complexes of alkali metal ions with poly(ethylene oxide). Polymer 14: 589. |
[7] | Armand MB, Chabagno JM, Duclot M (1978) Second Intern. Meeting on Solid Electrolytes, St. Andrews, Scotland, 20-22. |
[8] | Armand M (1983) Polymer solid electrolytes-an overview. Solid State Ionics 9-10(part 2): 745-754. |
[9] |
Papke BL, Ratner MA, Shriver DF (1982) Vibrational spectroscopic determination of structure and ion pairing in complexes of poly(ethylene oxide) with lithium salts. J Electrochem Society 129: 1434-1438. doi: 10.1149/1.2124179
![]() |
[10] |
Salleh NS, Aziz SB, Aspanut Z, et al. (2016) Electrical impedance and conduction mechanism analysis of bio polymer electrolytes based on methyl cellulose doped with ammonium iodide. Ionics 22: 2157-2167. doi: 10.1007/s11581-016-1731-0
![]() |
[11] | Agrawal RC, Sahu DK, Mahipal YK, et al. (2013) Ion transport property of Mg2+ ion conducting nano composite polymer electrolytes membranes: Study of effect of active/passive filler particle dispersal on conductivity. Indian J Pure App Phys 51: 320-323. |
[12] | Malhotra A, Varshney PK (2015) Future prospects of Polymer electrolytes. Int J Sci Res 4/10: 43-45. |
[13] |
Blonsky PM, Shriver DF, Austin P, et al. (1986) Complex formation and ionic conductivity of polyphosphazene solid electrolytes. Solid State Ionics 18-19: 258-264. doi: 10.1016/0167-2738(86)90123-2
![]() |
[14] |
Vincent CA (1987) Polymer electrolytes. Prog Solid State Chem 17: 145-261. doi: 10.1016/0079-6786(87)90003-3
![]() |
[15] |
Ratner MA, Nitzan A (1989) Conductivity in polymer ionics. Dynamic disorder and correlation. Faraday Discuss Chem Soc 88: 19-42. doi: 10.1039/dc9898800019
![]() |
[16] |
Borjesson L, Martin S, Torell L, et al. (1986) Sequential hypersonic dampings due to fast ion diffusion and structural relaxation in (AgI)x(AgPO3)1-x ionic liquids. Solid State Ionics 18-19: 141-146. doi: 10.1016/0167-2738(86)90101-3
![]() |
[17] |
Watanabe M, Sanui K, Ogata N, et al. (1985) Ionic conductivity and mobility in network polymers from poly(propylene oxide) containing lithium perchlorate. J Appl Phys 57: 123-128. doi: 10.1063/1.335386
![]() |
[18] |
Cameron GG, Ingram MD, Sarmouk K (1990) Conductivity and viscosity of liquid polymer electrolytes plasticized by propylene carbonate and tetrahydrofuran. Eur Polym J 26: 1097-1101. doi: 10.1016/0014-3057(90)90009-S
![]() |
[19] |
Bruce PG, Evans J, Vincent CA (1988) Conductivity and transference number measurements on polymer electrolytes. Solid State Ionics 28-30: 918-922. doi: 10.1016/0167-2738(88)90304-9
![]() |
[20] |
Chatani Y, Okamura S (1987) Crystal structure of poly(ethylene oxide)-sodium iodide complex. Polymer 28: 1815-1820. doi: 10.1016/0032-3861(87)90283-7
![]() |
[21] |
Abbrent S, Plestil J, Hlavata D, et al. (2001) Crystallinity and morphology of PVdF-HFP-based gel electrolytes. Polymer 42: 1407-1416. doi: 10.1016/S0032-3861(00)00517-6
![]() |
[22] |
Angell CA, Liu C, Sanchez E (1993) Rubbery solid electrolytes with dominant cationic transport and high ambient conductivity. Nature 362: 137-139. doi: 10.1038/362137a0
![]() |
[23] |
Quartarone E, Tomasi C, Mustarelli P, et al. (1998) Long-term structural stability of PMMA-based gel polymer electrolytes. Electrochim Acta 43: 1435-1439. doi: 10.1016/S0013-4686(97)10080-9
![]() |
[24] |
Prasadini KW, Perera KS, Vidanapathirana KP (2019) Characterization of an ionic liquid based gel polymer electrolyte for potential applications. J Natl Sci Found Sri Lanka 47: 133-138. doi: 10.4038/jnsfsr.v47i1.8930
![]() |
[25] |
Sanchez C, Belleville P, Popall M, et al. (2011) Applications of advanced hybrid organic-inorganic nanomaterials: from laboratory to market. Chem Soc Revs 40: 696-753. doi: 10.1039/c0cs00136h
![]() |
[26] |
Forsyth M, Porcarelli L, Wang X, et al. (2019) Innovative electrolytes based on ionic liquids and polymers for next generation solid state batteries. Acc Chem Res 52: 686-694. doi: 10.1021/acs.accounts.8b00566
![]() |
[27] | Armand M, Endres F, MacFarlane DR, et al. (2010) Ionic-liquid materials for the electrochemical challenges of the future. Mater Sustainable Energy 8: 129-137. |
[28] |
Le Bideau J, Viau L, Vioux A (2011) Ionogels, ionic liquid based hybrid materials. Chem Soc Rev 40: 907-925. doi: 10.1039/C0CS00059K
![]() |
[29] |
Ma F, Zhang Z, Yan W, et al. (2019) Solid polymer electrolyte based on polymerized ionic liquid for high performance All solid state lithium ion batteries. ACS Sustainble Chem Eng 7: 4675-4683. doi: 10.1021/acssuschemeng.8b04076
![]() |
[30] |
Sapri MNZM, Ahmed AH, Mahat MM, et al. (2017) Thermal analysis of 1ethyl3methylimmidazoliumtrifluoromethanesulfonate ionic liquid to PEO-NaCF3SO3 polymer electrolyte. Solid State Phenom 268: 338-342. doi: 10.4028/www.scientific.net/SSP.268.338
![]() |
[31] | Passerini S, Motanino M, et al. (2013) In: Mittal V Editor, Li polymer batteries based on ionic liquids in polymers for energy storage and conversion. John Wiley and Scri Verner Pub, USA. |
[32] |
Prasanna CMS, Suthanthiraraj SA (2016) Effective influences of 1-ethyl-3-methylimidazolium bis(trifluoromethanesulfonyl)imide (EMIMTFSI) ionic liquid on the ion transport properties of micro porous zinc ion conducting poly(vinyl chloride)/poly(ethyl methacrylate) blend based polymer electrolytes. J Polym Res 23: 140-157. doi: 10.1007/s10965-016-1043-0
![]() |
[33] |
Zhang S, Sun N, He X, et al. (2006) Physical Properties of Ionic Liquids: Database and Evaluation. J Phys Chem Ref Data 35: 1475-1517. doi: 10.1063/1.2204959
![]() |
[34] |
Yang P, Liu L, Li L, et al. (2014) Gel polymer electrolyte based on polyvinylidenefluoride-co-hexafluoropropylene and ionic liquid for lithium ion battery. Electrochim Acta 115: 454-460. doi: 10.1016/j.electacta.2013.10.202
![]() |
[35] |
Anandan S, Pitchumani S, Muthuraaman B, et al. (2006) Heteropolyacid-impregnated PVDF as a solid polymer electrolyte for dye-sensitized solar cells. Sol Energy Mater Sol Cells 90: 1715-1720. doi: 10.1016/j.solmat.2005.09.005
![]() |
[36] |
Noda A, Hayamizu K, Watanabe M (2001) Pulsed gradient spin echo 1H and 19F NMR ionic diffusion coefficient, viscosity and ionic conductivity of Non chloroaluminate room temperature ionic liquids. J Phys Chem B 105: 4603-4610. doi: 10.1021/jp004132q
![]() |
[37] |
Gerbaldi C, Nair JR, Ahmad S, et al. (2010) UV-cured polymer electrolytes encompassing hydrophobic room temperature ionic liquid for lithium batteries. J Power Sources 195: 1706-1713. doi: 10.1016/j.jpowsour.2009.09.047
![]() |
[38] |
Hayes R, Warr GG, Atkin R (2015) Structure and Nanostructure in Ionic Liquids. Chem Rev 115: 6357-6426. doi: 10.1021/cr500411q
![]() |
[39] |
Karunarathne KAJK, Perera KS, Vidanapathirana KP, et al. (2019) Fabrication and evaluation of an electrochemical double layer capacitor with natural graphite electrodes and magnesium trifluoromethane sulfonate based gel polymer electrolyte. J Solid State Electrochem 23: 2165-2171. doi: 10.1007/s10008-019-04309-2
![]() |
[40] |
Kobayashi Y, Shono K, Kobayashi T, et al. (2017) A long life 4 V class lithium-ion polymer battery with liquid-free polymer electrolyte. J Power Sources 341: 257-263. doi: 10.1016/j.jpowsour.2016.12.009
![]() |
[41] |
Syahidah SN, Majid SR (2015) Ionic liquid based polymer gel electrolytes for symmetrical solid state electrical double layer capacitor operated at different operating voltages. Electrochim Acta 175: 184-192. doi: 10.1016/j.electacta.2015.02.215
![]() |
[42] |
Missan HPS, Lalia BS, Karan K, et al. (2010) Polymer-ionic liquid nano-composites electrolytes: Electrical, thermal and morphological properties. Mater Sci Eng: B 175: 143-149. doi: 10.1016/j.mseb.2010.07.017
![]() |
[43] |
Dirican M, Yanilmaz M, Fu K, et al. (2014) Carbon-enhanced electrodeposited SnO2/carbon nanofiber composites as anode for lithium-ion batteries. J Power Sources 264: 240-247. doi: 10.1016/j.jpowsour.2014.04.102
![]() |
[44] |
Choi JA, Kim SH, Kim DW (2010) Enhancement of thermal stability and cycling performance in lithium-ion cells through the use of ceramic-coated separators. J Power Sources 195: 6192-6196. doi: 10.1016/j.jpowsour.2009.11.020
![]() |
[45] | Rosdi A, Zainol NH, Osman Z (2016) Ionic transport and Electrochemical stability of PVdF-HFP based gel polymer electrolytes. Int Symp Front Appl Phys, 0500031-05000356. |
[46] |
Gupta H, Balo SL, Singh VK, et al. (2017) Effect of temperature on electrochemical performance of ionic liquid based polymer electrolyte with Li/LiFePO4 electrodes. Solid State Ionics 309: 192-199. doi: 10.1016/j.ssi.2017.07.019
![]() |
[47] |
Kim JI, Choi Y, Chung KY, et al. (2017) A structurable gel-polymer electrolyte for sodium ion batteries. Adv Funct Mater 27: 1701768. doi: 10.1002/adfm.201701768
![]() |
[48] |
Gong SD, Huang Y, Cao HJ, et al. (2016) A green and environment-friendly gel polymer electrolyte with higher performances based on the natural matrix of lignin. J Power Sources 307: 624-633. doi: 10.1016/j.jpowsour.2016.01.030
![]() |
[49] |
Aziz SB, Woo TJ, Kadir MFZ, et al. (2018). A conceptual review on polymer electrolytes and ion transport models. J Sci: Adv Mater Devices 3: 1-17. doi: 10.1016/j.jsamd.2018.01.002
![]() |
[50] |
Zarrougui R, Dhahbi M, Lemordant D (2010) Effect of temperature and composition on the transport and thermodynamic properties of binary mixtures of ionic liquid N-Butyl-N-methylpyrrolidinium bis(Trifluoromethanesulfonyl)imide and propylene carbonate. J Solution Chem 39: 921-942. doi: 10.1007/s10953-010-9562-5
![]() |
[51] |
Choudhury NS, Patterson JW (1970) Steady-State chemical potential profiles in solid electrolytes. J Electrochem Soc 117: 1384-1388. doi: 10.1149/1.2407327
![]() |
[52] |
Liew CW, Ramesh S, Ramesh K, et al. (2012) Preparation and characterization of lithium ion conducting ionic liquid-based biodegradable corn starch polymer electrolytes. J Solid State Electrochem 16: 1869-1875. doi: 10.1007/s10008-012-1651-5
![]() |
[53] |
Weston J, Steele B (1982) Effects of inert fillers on the mechanical and electrochemical properties of lithium salt-poly(ethylene oxide) polymer electrolytes. Solid State Ionics 7: 75-79. doi: 10.1016/0167-2738(82)90072-8
![]() |
[54] | Kumar SR, Aparna Y (2016) Synthesis and characterization of PEO complexed with NaClO4soluble base salt and Nb2O5 Nano filler. Int J Engi Res Sci 2: 16-24. |
[55] |
Khanmirzaei MH, Ramesh S (2014) Nanocomposite polymer electrolyte based on rice starch/ionic liquid/TiO2 nano particles for solar cell application. Measurement 58: 68-72. doi: 10.1016/j.measurement.2014.08.009
![]() |
[56] |
Li GC, Zhang P, Zhang HP, et al. (2008) A porous polymer electrolyte based on P(VDF-HFP) prepared by a simple phase separation process. Electrochem Commun 10: 1883-1885. doi: 10.1016/j.elecom.2008.09.035
![]() |
[57] |
Ortega PFR, Trigueiro JPC, Silva GG, et al. (2016) Improving supercapacitor capacitance by using a novel gel nanocomposite polymer electrolyte based on nanostructured SiO2, PVDF and imidazolium ionic liquid. Electrochim Acta 188: 809-817. doi: 10.1016/j.electacta.2015.12.056
![]() |
[58] |
Deka M, Kumar A (2011) Electrical and electrochemical studies of poly(vinylidene fluoride)-clay nanocomposite gel polymer electrolytes for Li-ion batteries. J Power Sources 196: 1358-1364. doi: 10.1016/j.jpowsour.2010.09.035
![]() |
[59] |
Ngai KS, Ramesh S, Ramesh K, et al. (2016) A review of polymer electrolytes: fundamental, approaches and applications. Ionics 22: 1259-1279. doi: 10.1007/s11581-016-1756-4
![]() |
[60] |
Lim JY, Kang DA, Kim NU, et al. (2019) Bicontinuously crosslinked polymer electrolyte membranes with high ion conductivity and mechanical strength. J Membr Sci 589: 117250-117256. doi: 10.1016/j.memsci.2019.117250
![]() |
[61] |
Gong C, Xue Z, Wang X, et al. (2014) Poly(ethylene glycol) grafted multi-walled carbon nanotubes/LiFePO4 composite cathodes for lithium ion batteries. J Power Sources 246: 260-268. doi: 10.1016/j.jpowsour.2013.07.091
![]() |
[62] |
Jiang G, Maeda S, Yang H, et al. (2005) All solid-state lithium-polymer battery using poly(urethane acrylate)/nano-SiO2 composite electrolytes. J Power Sources 141: 143-148. doi: 10.1016/j.jpowsour.2004.09.004
![]() |
[63] |
Wang X (2003) Novel composite polymer electrolytes based on poly(ether-urethane) network polymer and modified montmorillonite. Electrochem Commun 5: 1025-1029. doi: 10.1016/j.elecom.2003.09.018
![]() |
[64] |
Kim SK, Choi SW, Jeon WS, et al. (2012) Cross-Linked benzoxazine-benzimidazole copolymer electrolyte membranes for fuel cells at elevated temperature. Macromolecule 45: 1438-1446. doi: 10.1021/ma202694p
![]() |
[65] |
Zhang P, Zhang HP, Li GC, et al. (2008) A novel process to prepare porous membranes comprising SnO2 nanoparticles and P(MMA-AN) as polymer electrolyte. Electrochem Commun 10: 1052-1055. doi: 10.1016/j.elecom.2008.04.037
![]() |
[66] |
Ishikawa M, Sugimoto T, Kikuta M, et al. (2006) Pure ionic liquid electrolytes compatible with a graphitized carbon negative electrode in rechargeable lithium-ion batteries. J Power Sources 162: 658-662. doi: 10.1016/j.jpowsour.2006.02.077
![]() |
[67] |
Kumar D, Hashmi SA (2010) Ionic liquid based sodium ion conducting gel polymer electrolytes. Solid State Ionics 181: 416-423. doi: 10.1016/j.ssi.2010.01.025
![]() |
[68] |
Rennie AJR, Ramirez NS, Toressi RM, et al. (2013) Ether bond containing ionic liquids as supercapacitor electrolytes. J Phys Chem Lett 4: 2970-2974. doi: 10.1021/jz4016553
![]() |
[69] |
Song D, Chen Z, Cui P, et al. (2015) NH3-treated WO3 as low-cost and efficient counter electrode for dye-sensitized solar cells. Nanoscale Res Lett 10: 1-6. doi: 10.1186/1556-276X-10-1
![]() |
[70] |
Song D, Cui P, Wang T, et al. (2016) Bunchy TiO2 hierarchical spheres with fast electron transport and large specific surface area for highly efficient dye-sensitized solar cells. Nano Energy 23: 122-128. doi: 10.1016/j.nanoen.2016.03.006
![]() |
[71] |
Zhou Z, Wang Y, Xu D, et al. (2010) Fabrication of Cu2ZnSnS4 screen printed layers for solar cells. Sol Energy Mater Sol Cells 94: 2042-2045. doi: 10.1016/j.solmat.2010.06.010
![]() |
[72] |
Singh PK, Bhattacharya B, Mehra RM, et al. (2011) Plasticizer doped ionic liquid incorporated solid polymer electrolytes for photovoltaic application. Curr Appl Phys 11: 616-619. doi: 10.1016/j.cap.2010.10.012
![]() |
[73] |
Luo J, Hu J, Saak W, et al. (2011) Protic ionic liquid and ionic melts prepared from methanesulfonic acid and 1H-1,2,4-triazole as high temperature PEMFC electrolytes. J Mater Chem 21: 10426-10436. doi: 10.1039/c0jm04306k
![]() |
[74] |
Díaz M, Ortiz A, Vilas M, et al. (2014) Performance of PEMFC with new polyvinyl-ionic liquids based membranes as electrolytes. Int J Hydrogen Energy 39: 3970-3977. doi: 10.1016/j.ijhydene.2013.04.155
![]() |
[75] |
Takegawa A, Murakami M, Kaneko Y, et al. (2010) Preparation of chitin/cellulose composite gels and films with ionic liquids. Carbohydr Polym 79: 85-90. doi: 10.1016/j.carbpol.2009.07.030
![]() |
[76] |
Ning W, Xingxiang Z, Haihui L, et al. (2009) 1-Allyl-3-methylimidazolium chloride plasticized-corn starch as solid biopolymer electrolytes. Carbohydr Polym 76: 482-484. doi: 10.1016/j.carbpol.2008.11.005
![]() |
[77] |
Du Z, Su Y, Qu Y, et al. (2019) A mechanically robust, biodegradable and high performance cellulose gel membrane as gel polymer electrolyte of lithium-ion battery. Electrochim Acta 299: 19-26. doi: 10.1016/j.electacta.2018.12.173
![]() |
[78] |
Ye YS, Rick J, Hwang BJ (2013) Ionic liquid polymer electrolytes. J Mater Chem A 1: 2719-2743. doi: 10.1039/C2TA00126H
![]() |
[79] |
Wang X, Girard GM, Zhu H, et al. (2019) Poly(ionic liquid)/electrospun nanofibre composite polymer electrolytes for high energy density and safe Li metal batteries. ACS Appl Energy Mat 2: 6237-6245. doi: 10.1021/acsaem.9b00765
![]() |
[80] |
George A, Brandt A, Tran K, et al. (2015) Design of low-cost ionic liquids for lignocellulosic biomass pretreatment. Green Chem 17: 1728-1734. doi: 10.1039/C4GC01208A
![]() |
[81] |
Shen SY, Dong RX, Shih PT, et al. (2014) Novel polymer gel electrolyte with organic solvents for quasi-solid-state dye-sensitized solar cells. ACS Appl Mater Interfaces 6: 18489-18496. doi: 10.1021/am505394v
![]() |
[82] |
Lu Q, Fu J, Chen L, et al. (2019) Polymeric polyhedral oligomeric silsesquioxane ionic liquids based solid polymer electrolytes for lithium ion batteries. J Power Sources 414: 31-40. doi: 10.1016/j.jpowsour.2018.12.085
![]() |
[83] |
Yang K, Liao Z, Zhang Z, et al. (2019) Ionic plastic crystal-polymeric ionic liquid solid-state electrolytes with high ionic conductivity for lithium ion batteries. Mater Lett 236: 554-557. doi: 10.1016/j.matlet.2018.11.003
![]() |
[84] |
Li X, Zhang W, Cai J, et al. (2019) Hierarchical nanosheets constructed by integration of bimetallic sulfides into N-Doped carbon: Enhanced diffusion kinetics and cycling stability for sodium storage. Nano Energy 62: 239-249. doi: 10.1016/j.nanoen.2019.05.040
![]() |
[85] |
Li X, Wu G, Liu X, et al. (2017) Orderly integration of porous TiO2 (B) nanosheets into bunchy hierarchical structure for high-rate and ultralong-lifespan lithium-ion batteries. Nano Energy 31: 1-8. doi: 10.1016/j.nanoen.2016.11.002
![]() |
1. | Daria Nikolaeva, Katrien Verachtert, Itxaso Azcune, Johannes C. Jansen, Ivo F.J. Vankelecom, Influence of ionic liquid-like cationic pendants composition in cellulose based polyelectrolytes on membrane-based CO2 separation, 2021, 255, 01448617, 117375, 10.1016/j.carbpol.2020.117375 | |
2. | Sebastián Bonardd, Alejandro Ángel, Ángel Norambuena, Deysma Coll, Alain Tundidor-Camba, Pablo A. Ortiz, Novel Polyelectrolytes Obtained by Direct Alkylation and Ion Replacement of a New Aromatic Polyamide Copolymer Bearing Pyridinyl Pendant Groups, 2021, 13, 2073-4360, 1993, 10.3390/polym13121993 | |
3. | Kajari Chatterjee, M.K. Sridhar, Akhilesh Kumar Singh, Kisor Kumar Sahu, 2023, Chapter 3, 978-1-80356-221-6, 10.5772/intechopen.107938 | |
4. | Pankaj Singh, A L Saroj, Effect of ionic liquid on structural, thermal and electrical transport properties of PVA-PVP based polymer blend electrolyte membrane, 2021, 96, 0031-8949, 115701, 10.1088/1402-4896/ac12e8 | |
5. | F. F. Awang, M. F. Hassan, K. H. Kamarudin, Investigation of structural and electrical properties of a biopolymer materials with its potential application in solid-state batteries, 2023, 80, 0170-0839, 1463, 10.1007/s00289-022-04124-2 | |
6. | Huizhe Niu, Minling Ding, Nan Zhang, Xulong Guo, Ping Guan, Xiaoling Hu, Ionic Liquid‐Modified Silicon Nanoparticles Composite Gel Polymer Electrolyte for High‐Performance Lithium Batteries, 2023, 10, 2196-0216, 10.1002/celc.202201015 | |
7. | Flávia C.A. Silva, Paulo F.R. Ortega, Rodrigo A. dos Reis, Rodrigo L. Lavall, Luciano T. Costa, Polymer-ion interactions in PVDF@ionic liquid polymer electrolytes: A combined experimental and computational study, 2022, 427, 00134686, 140831, 10.1016/j.electacta.2022.140831 | |
8. | N.F. Mazuki, M.Z. Kufian, Mohd Mawardi Saari, A.S. Samsudin, Influencing of [EDIMP]TFSI in PMMA-PLA doped LiTFSI based hybrid gel polymer electrolyte on the variation in crystallinity phase and ionic conduction properties, 2023, 621, 00223093, 122634, 10.1016/j.jnoncrysol.2023.122634 | |
9. | L. P. Safonova, L. E. Shmukler, Polymer Electrolytes Based on Polybenzimidazole, Poly(Vinylidene Fluoride-co-Hexafluoropropylene), and Ionic Liquids, 2023, 65, 0965-545X, 312, 10.1134/S0965545X23701080 | |
10. | Kumudu S. Perera, Kamal P. Vidanapathirana, Lewis J. Adams, Chris S. Hawes, Nilanthy Balakrishnan, Symmetric double-layer capacitor with natural rubber and sodium salt-based solid polymer electrolyte and reduced graphene oxide electrodes, 2024, 97, 2352152X, 112683, 10.1016/j.est.2024.112683 | |
11. | Nur Aainaa, Suhaila Abdullah, Norazlina Hashim, Badrul Haswan Besar, Nurul Anniyah Mohamad Sobri, Lili Shakirah Hassan, 2024, 3027, 0094-243X, 050004, 10.1063/5.0207027 | |
12. | Jiajia Song, Lingjiang Kou, Yong Wang, Taotao Ai, Wenhu Li, Koji Kajiyoshi, Panya Wattanapaphawong, Yi Li, Synthesis and electrochemical properties of hydrangea‐like (NH4)2V4O9/V5O12·6H2O cathode for zinc‐ion battery, 2024, 21, 1546-542X, 319, 10.1111/ijac.14509 | |
13. | L. P. Safonova, L. E. Shmukler, Polymer Electrolytes Based on Polybenzimidazole, Poly(Vinylidene Fluoride-co-Hexafluoropropylene), and Ionic Liquids, 2023, 65, 2412-9844, 249, 10.31857/S2308112023700566 |
Method | Properties | Merits | Demerits |
Changing component concentration | Mechanical properties may change; Liquid nature may arise | Thickness can be reduced | Conductivity may reduce; Difficulty in handling |
Adding inorganic nano particles | Thick film | Reduction of reactivity Increase mechanical strength | Cost may be high |
Blending | Combined properties | Many desirable properties are present simultaneously | Needs correct methods for blending |
Method | Properties | Merits | Demerits |
Changing component concentration | Mechanical properties may change; Liquid nature may arise | Thickness can be reduced | Conductivity may reduce; Difficulty in handling |
Adding inorganic nano particles | Thick film | Reduction of reactivity Increase mechanical strength | Cost may be high |
Blending | Combined properties | Many desirable properties are present simultaneously | Needs correct methods for blending |