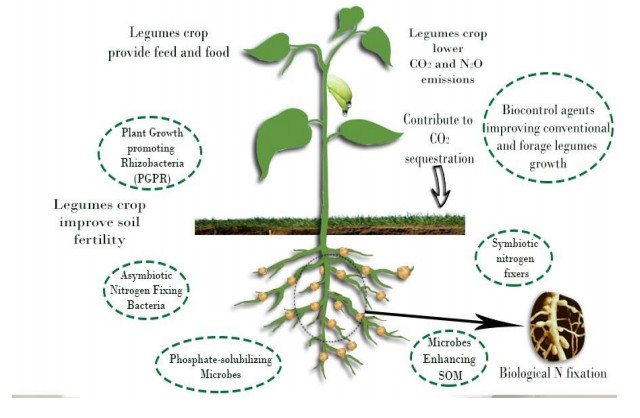
The diverse community of endophyte and rhizobacteria is a critical resource in enhancing plant growth and resistance against abiotic and biotic stress. These microbes include various bacterial communities dominated by Proteobacteria, Bacteroidetes, Actinobacteria and Firmicutes. They inhabit and proliferate in plant tissues forming beneficial associations compared to other microbes residing in the exospheric region. Despite the demonstration of the presence of bacterial endophytes in crops, their role in supporting nutrient bioavailability and acquisition in degraded soils is largely unexplored. In addition, the practical application of these microbial communities in the field has not been demonstrated. A comprehensive understanding of plant-endophyte interactions will help restore degraded soils and plant nutrient acquisition in resource-limiting field environments. Anthropogenic farming practices such as the use of chemical fertilizers to restore degraded soils have proved to be detrimental to soil structure, function and soil biodiversity. Recent studies in soil and root structure suggest that the rhizosphere and endophytic bacterial communities could potentially be used to enhance crop production. Other studies have shown that endophytic microbes play a key role in modulation of metabolism in plants, stimulation of plant growth, and aid in plant adaptation to environmental stress using phytohormone signaling. The use of rhizosphere and endophytic bacteria can significantly reduce the amount of agrochemicals that contribute to environmental pollution. In the context of the changing climatic conditions, some beneficial rhizospheric and endophytic bacterial communities enhance adaptation and resilience, thereby promoting sustainable farming systems. The current review addresses the concepts, challenges, and roles of the bacterial endophytes and rhizobacteria as components of the plant microbiota, and their prospective use in reclamation of degraded soil environments.
Citation: Simon Wambui Mburu, Gilbert Koskey, Ezekiel Mugendi Njeru, John M. Maingi. Revitalization of bacterial endophytes and rhizobacteria for nutrients bioavailability in degraded soils to promote crop production[J]. AIMS Agriculture and Food, 2021, 6(2): 496-524. doi: 10.3934/agrfood.2021029
[1] | Sonia Maria Lima Santos do Vale, Amauri Siviero, Lauro Saraiva Lessa, Eduardo Pacca Luna Mattar, Paulo Arthur Almeida do Vale . Biotechnological potential of endophytic bacteria of bamboo Guadua sp. for promotion of growth of micropropagated yam plants (Dioscorea rotundata Poir). AIMS Agriculture and Food, 2020, 5(4): 850-867. doi: 10.3934/agrfood.2020.4.850 |
[2] | Boris Boincean, Amir Kassam, Gottlieb Basch, Don Reicosky, Emilio Gonzalez, Tony Reynolds, Marina Ilusca, Marin Cebotari, Grigore Rusnac, Vadim Cuzeac, Lidia Bulat, Dorian Pasat, Stanislav Stadnic, Sergiu Gavrilas, Ion Boaghii . Towards Conservation Agriculture systems in Moldova. AIMS Agriculture and Food, 2016, 1(4): 369-386. doi: 10.3934/agrfood.2016.4.369 |
[3] | Murimi David Njue, Mucheru-Muna Monicah Wanjiku, Mugi-Ngenga Esther, Zingore Shamie, Mutegi James Kinyua . Nutrient management options for enhancing productivity and profitability of conservation agriculture under on-farm conditions in central highlands of Kenya. AIMS Agriculture and Food, 2020, 5(4): 666-680. doi: 10.3934/agrfood.2020.4.666 |
[4] | Saowanee Wijitkosum, Preamsuda Jiwnok . Effect of biochar on Chinese kale and carbon storage in an agricultural area on a high rise building. AIMS Agriculture and Food, 2019, 4(1): 177-193. doi: 10.3934/agrfood.2019.1.177 |
[5] | Methuselah Mang’erere Nyamwange, Ezekiel Mugendi Njeru, Monicah Mucheru-Muna, Felix Ngetich . Soil management practices affect arbuscular mycorrhizal fungi propagules, root colonization and growth of rainfed maize. AIMS Agriculture and Food, 2018, 3(2): 120-134. doi: 10.3934/agrfood.2018.2.120 |
[6] | W. Mupangwa, I. Nyagumbo, E. Mutsamba . Effect of different mulching materials on maize growth and yield in conservation agriculture systems of sub-humid Zimbabwe. AIMS Agriculture and Food, 2016, 1(2): 239-253. doi: 10.3934/agrfood.2016.2.239 |
[7] | Milka N. Kiboi, Felix K. Ngetich, Daniel N. Mugendi . Nitrogen budgets and flows in African smallholder farming systems. AIMS Agriculture and Food, 2019, 4(2): 429-446. doi: 10.3934/agrfood.2019.2.429 |
[8] | Johan Sukweenadhi, Eloqui Viectorica Wiranata, Ida Bagus Made Artadana, Kang-Se Chang . Isolation and in vitro screening of plant growth promoting bacteria from rhizosphere and root tissues of potato tuber (Solanum tuberosum L.). AIMS Agriculture and Food, 2023, 8(4): 1028-1037. doi: 10.3934/agrfood.2023055 |
[9] | Janice Liang, Travis Reynolds, Alemayehu Wassie, Cathy Collins, Atalel Wubalem . Effects of exotic Eucalyptus spp. plantations on soil properties in and around sacred natural sites in the northern Ethiopian Highlands. AIMS Agriculture and Food, 2016, 1(2): 175-193. doi: 10.3934/agrfood.2016.2.175 |
[10] | Emmanuel Hanyabui, Samuel Obeng Apori, Kwame Agyei Frimpong, Kofi Atiah, Thomas Abindaw, Muhammed Ali, Joshua Yeboah Asiamah, John Byalebeka . Phosphorus sorption in tropical soils. AIMS Agriculture and Food, 2020, 5(4): 599-616. doi: 10.3934/agrfood.2020.4.599 |
The diverse community of endophyte and rhizobacteria is a critical resource in enhancing plant growth and resistance against abiotic and biotic stress. These microbes include various bacterial communities dominated by Proteobacteria, Bacteroidetes, Actinobacteria and Firmicutes. They inhabit and proliferate in plant tissues forming beneficial associations compared to other microbes residing in the exospheric region. Despite the demonstration of the presence of bacterial endophytes in crops, their role in supporting nutrient bioavailability and acquisition in degraded soils is largely unexplored. In addition, the practical application of these microbial communities in the field has not been demonstrated. A comprehensive understanding of plant-endophyte interactions will help restore degraded soils and plant nutrient acquisition in resource-limiting field environments. Anthropogenic farming practices such as the use of chemical fertilizers to restore degraded soils have proved to be detrimental to soil structure, function and soil biodiversity. Recent studies in soil and root structure suggest that the rhizosphere and endophytic bacterial communities could potentially be used to enhance crop production. Other studies have shown that endophytic microbes play a key role in modulation of metabolism in plants, stimulation of plant growth, and aid in plant adaptation to environmental stress using phytohormone signaling. The use of rhizosphere and endophytic bacteria can significantly reduce the amount of agrochemicals that contribute to environmental pollution. In the context of the changing climatic conditions, some beneficial rhizospheric and endophytic bacterial communities enhance adaptation and resilience, thereby promoting sustainable farming systems. The current review addresses the concepts, challenges, and roles of the bacterial endophytes and rhizobacteria as components of the plant microbiota, and their prospective use in reclamation of degraded soil environments.
Over the years, crop production in smallholder farming systems has steadily declined due to the diminishing soil fertility, change in climatic conditions, unavailability of additional arable land, and overdependence on rainwater for farming [1]. Agricultural land degradation caused by anthropogenic activities such as excessive use of inorganic fertilizers and pesticides, continuous cultivation of exhaustive crops such as cereals, and the emergence of destructive phytopathogens have contributed to the decline in crop productivity. According to Kopittke et al. [2], the leading cause of soil degradation is the loss of soil fertility which becomes a risk factor to crop production. Moreover, Singh et al. [3] indicated that the advances in sustainable crop production have been challenged mainly by soil degradation, depletion of soil organic matter, demographic development, and climate change. Since the green revolution period, crop production has been characterized by the use of chemical fertilizers, heavy farm machineries, intensive tillage, high-yielding crop varieties, and use of pesticides that are recalcitrant to biodegradation. However, these practices have ruined soil ecology and disrupted the key plant-microbe interactions beneficial in soil fertility restoration [4]. The use of beneficial biological agents and their derivatives in sustainable agriculture is gaining popularity with the recent advances in molecular technology. This is because they are eco-friendly, versatile, and can enhance crop productivity in a wide range of environments despite the changing climatic conditions [5].
The microbial component in the plant endosphere and rhizosphere form beneficial associations with plants that can improve crop productivity [6]. They promote plant resilience to various abiotic and biotic factors that limit growth and production [7]. These microbes can live either internally or externally on the host plant tissues. For instance, rhizospheric bacteria inhabit plant roots within the soil, and epiphytic bacteria inhabit plant leaf surfaces. Meanwhile, bacterial endophytes are found inside the host plants [5]. These bacterial types create complex relationships with the host plants where they act as plant growth promoters (Figure 1). The role of plant-associated bacteria in enhancing crop production and soil fertility has been widely studied [8]. Outstandingly, there is scanty data in the literature concerning field-based research investigating the role of bacterial endophytes in the restoration of degraded soils and crop production. On degraded soils, it has not been possible to replicate on the actual field conditions the successes of plant-endophytic interactions seen in laboratory experiments.
Rhizobacteria refer to plant growth-promoting bacteria that exist in the rhizosphere. The rhizosphere consists of the narrow zone of soil influenced by plant root system where maximum microbial activities occurs [9]. The rhizosphere zone is an ecological niche that provides a rich source of nutrients and energy for plant growth. The rhizobacteria are abundant plant partners in the rhizosphere, but they differ in their roles in plant growth promotion. Various interactions occur between plants and rhizobacteria in the rhizosphere. These interactions are equally important, and involve of signals between the rhizobacteria and the plant roots that regulate their biochemical activities [10]. The rhizobacteria are crucial in the rhizosphere for nutrients cycling, carbon sequestration, and ecosystem functioning that promote plant growth, yield and nutrition. Various bacteria genera have been utilized as plant growth-promoting rhizobacteria (PGPR) and include Burkholderia, Pseudomonas, Arthrobacter, Bacillus, Serratia, Micrococcus, Chromobacterium, Erwinia, Azospirillum, Caulobacter, Agrobacterium, and Azotobacter [9]. The rhizobacteria produce plant growth modulating phytohormones such as ethylene, gibberellins, and auxins. Other important metabolites include production of siderophores, enzymes, organic acids, antibiotics, biosurfactants, nitric oxide, and osmolytes. The metabolites are responsible for improved nutrients uptake, tolerance to abiotic stress, nitrogen fixation, suppression of pathogenic organisms [11].
Bacterial endophytes are also referred to as plant growth-promoting rhizobacteria (PGPR) and are believed to be part of the group of bacteria that occupy the rhizosphere [12]. Some studies have defined bacterial endophytes as bacteria that do not harm the plant but can be isolated inside surface-sterilized plant materials [13]. These bacteria have adaptive abilities to invade and colonize their host [13]. Endophytic bacteria enhance growth by establishing synergistic interactions with the host plant or antagonistic interactions with soil pathogens [14]. These inter-microbe and plant-microbe interactions play a crucial role in the restoration of degraded land into an agriculturally productive landscape through various complex biochemical mechanisms. Moreover, bacterial endophytes can potentially exert greater beneficial effects on the host plants compared to other plant-associated bacteria. It has been reported that bacterial endophytes have undergone a longer evolutionary selection over many generations and are well adapted to the host plant [15]. In addition, they are inherited and can be transferred through seeds, making them more compatible and effective in inducing plant growth promotion [7]. This inheritability factor is important in selecting adaptive and effective endophytes associated with a specific plant of agricultural importance, especially in plant breeding and tackling climate change-related challenges. Their capacity to tolerate and induce resilience against biotic and abiotic stresses in plants can be exploited to solve edaphic and pathogen-related challenges facing the crop production sector. According to Pandey et al. [16], various benefits associated with endophytes can be more conspicuous when the plants are subjected to adverse environmental stress. Habitat-imposed stress triggers plant-microbial signaling, which forms a complex communication pathway that promotes ecological interactions between individual organisms leading to better adaptation of the host plant to the disturbed environment. Endophytic bacteria enable their host to outcompete other plants such as weeds by enhancing the production of allelopathic biomolecules with antagonistic effects against competitors [17]. Notably, host plants are also able to "choose" their microbial partners that enable them to gain maximum benefits from the interactions [18].
Bacterial endophytes affect host plant development positively without any noticeable harm while suppressing any pathogen that may invade the plant [19]. In return, the endophytic microbes benefit and use the plant endosphere as a unique and safe haven that is unperturbed by the harsh climatic conditions that could harm and affect their functionality [20]. Besides, most endophytic bacteria exhibit a biphasic life cycle where they alternate between the soil and plant environment, thus surviving between seasons [21]. Other bacteria establish symbiotic structures such as nodules from legumes that harbour diverse bacterial strains. Interestingly, only the rhizobia responsible for nitrogen fixation are well known, while other bacterial endophytes are poorly studied [22]. Some studies on root nodule microbiome have shown more complex nodule microbial occupants than expected [23,24,25].
Soil rhizosphere harbors an active, diverse, and complex bacterial community associated with plants. Even though bacterial endophytes have been isolated in most of the plants studied, their adaptive traits in plant tissues are poorly known [29]. To study the diversity of bacterial endophytes, molecular and cultivation-based approaches have been used. It has been reported that bacterial endophytes could be more diverse than what is actually revealed from laboratory cultivation [30]. Bacteria endophytes comprise both cultivable and uncultivable species [31], which are further characterized as endophytes and endosymbionts. The use of culture-dependent and advanced molecular tools such as next-generation sequencing and metagenomics analyses have immensely enhanced the understanding of their functional diversity, composition, taxonomic and genetic diversity [32]. Sarhan et al. [33] prefer studying the diversity of bacterial endophytes using culturomics (culture-dependent techniques) since they can further be subjected to bioassays to establish their effect in promoting plant growth. Cultivation media that supports a wide range of endophytes remains undiscovered. Research efforts should be channeled to unfold the basic metabolic requirements and physiological functioning of various endophytes.
Metagenomics techniques involve the use of sequencing tools to analyze the genes for the entire population using extracted DNA [32]. However, during sequencing, one can opt for a specific phylogenetic marker such as the 16S rRNA gene, sequencing of internal transcribed spacer regions (ITS), or the entire genome [34] (Figure 2). In other words, the use of the metagenomics approach has allowed in-depth analyses of bacterial endophyte diversity. For instance, metagenomics has uncovered a hidden potential of rice endo-rhizosphere bacterial community whose endophytic competence might be crucial for stimulating plant growth and enhancing rice yield [35].
At least 200 bacterial genera or 16 phyla have been documented as endophytes from various plant hosts [22,36]. The most dominant bacterial endophytes phyla are Proteobacteria, Actinobacteria, Bacteroidetes, Cyanobacteria, Aguificae, Firmicutes, and Acidobacteria [37,38]. Among them, the most studied and prominent endophytic bacteria genera include members of Bacillus, Pseudomonas, Stenotrophomonas, Enterobacter, Burkholderia, and Azoarcus belonging to Proteobacteria phyla [9,39].
Liotti et al. [40] noted that it is advantageous to focus on both the diversity and species richness to comprehend the distribution and role of bacterial endophytes in their ecological niche. Similarly, Harrison and Griffin [41] indicated that a good diversity index should include information and dominance indices such as Simpson Index and Shannon-Wiener Index respectively, especially for the rhizospheric PGPR [42]. For instance, Andreolli et al. [43] revealed a higher diversity of bacterial endophytes from grapevines (Vitis vinifera cv. Corvina) aged 3–15 years. Interestingly, younger grapes had a greater genus richness [43]. Meanwhile, Koskey et al. [44] reported a high genetic diversity of endophytic rhizobia symbionts associating with Phaseolus vulgaris L. based on Shannon-Wiener and Simpson diversity indices [45]. Thus, there seems to be a complex diversity of the endophytic microbiome and with the advancing technological tools, further exploration could yield a huge bacterial diversity whose composition potential could have novel functionalities in transforming the current crop production.
Currently, the study of the functional diversity of endophytic bacteria is gaining attention, and various experiments are being undertaken to understand their functional roles in delivering agroecosystem services to plants and their co-existence with other PGPRs. Microbial functional diversity in an ecosystem refers to the various functional traits of microorganisms coexisting in a given ecosystem [46]. Studies have shown that functional diversity can dictate the nutrient balance, stability, dynamics, and productivity of the ecosystem [47]. Practically, plants would be more susceptible to environmental stress and less fit to withstand phytopathogens in the absence of the associated beneficial bacterial endophytes. However, it is a rare exception in any natural environment to find an endophyte-free plant since they have been reported in every studied plant species. Increased microbial functional diversity positively influences plant traits through the enhanced provision of agroecosystem services associated with soil fertility, productivity and overall plant health [13]. Prasannakumar et al. [48] applied metagenomics tools to study endophytic functional diversity associated with two finger millet varieties and reported several genes with functional roles in inducing resilience against blast disease caused by ascomycete fungus. Therefore, it is evident that the analysis of bacterial endophytes based on metagenomics techniques should focus more on the functionality of the genes to understand their roles compared to the sequencing of the core genes.
Endophytic diversity and their ability to colonize the host is strongly influenced by the environmental factors and host plant genotype [49]. Distinctively, the type of plant material used and the plant growth stage have been shown to influence the composition of bacterial endophytes present [50]. Hong et al. [51] carried out metagenomics analyses at different plant ages and reported a high variation of PGPRs, including endophytes, colonizing a 3-year old Panax ginseng plant. Different plant species have been reported to have different bacterial endophytes despite growing in the same environment [52]. This was also observed by Ding et al. [53] who reported that host plant species followed by sampling time and location of the plant materials are the most important determinants when selecting an endophytic community. Their distribution along the plant tissues could also differ with some endophytes concentrating on the roots while others on the stem and leaves [5]. Vertical evolutionary inheritance through seeds or stem cuttings also affects the predominant endophytic bacterial species present. Besides, the type of soil used to grow the plants also affects endophytic diversity [54]. This means the same plant species could have very diverse bacterial endophytes while growing in different agricultural soils. For instance, Rashid et al. [55] isolated different bacterial endophytes from one tomato cultivar that was grown in 15 different agricultural soils. Hence, the determinants of endophytic diversity seem to be complex and influenced by dynamic processes involving the environment, bacteria and the host plant. Further investigations on endophytic transcriptomics and determination of their distribution in the ecosystem and within the plant cell could reveal the key drivers needed for optimal plant-microbe-environment interactions.
Soil degradation is described as the decline in soil quality that is characterized by the reduction of valuable services and functions of the ecosystem. It is characterized by leaching and depletion of essential nutrients N and P, Ca2+ and Mg2+ deficiencies, increased Mn2+ and Al2+ toxicities, cation exchange capacity (CEC) reduction, salinization, poor water holding capacity, and soil acidification [56]. Soil degradation is also associated with the loss of soil biodiversity, reduction in organic carbon (OC), organic matter (OM), and the capacity to sink carbon (C) [57]. Soil degradation results in the loss of key functions of the soil ecosystem, therefore, reducing food supply, which may contribute to a surge of "environmental refugees" [58].
Land degradation is a global challenge facing agriculture. It is often termed a "silent disaster" due to its unnoticeable impacts leading to the decline of soil fertility and crop productivity. Approximately 24% of the productive soils worldwide is under consistent degradation while 40% of agricultural land is already degraded [59]. The degradation is majorly attributed to human-induced processes. For instance, the key drivers of soil degradation in agricultural land in sub-Saharan Africa (SSA) include excessive tillage, crop residue removal, unbalanced use of chemical fertilizers, poor crop cycle planning, and lack of sustainable soil fertility inputs [60,61]. This is compounded by excessive tillage, deforestation, and overgrazing, resulting in soil erosion and leaching of essential macro and micronutrients, hence affecting land productivity [56]. Additionally, chemical attributes such as salinity and waterlogging account for the degradation of agricultural lands. Approximately 80% of the SSA farms constitute smallholder farms characterized by limited farming area, insufficient farm inputs and lack of knowledge and necessary skills for crop production. Soil degradation in SSA has contributed to low agricultural productivity and poverty in rural communities, leading to rural-urban migration and land abandonment, which leads to food insecurity.
In this regard, soil degradation is a major threat that requires special attention to establish alternative soil management interventions that can reinstate the productivity of degraded soils. According to Chaer et al. [62], there is a correlation between soil degradation and quantitative analysis of soil microbial community. The size, composition and functionality of bacterial endophytes and other rhizospheric microbes can lessen the negative impacts of anthropogenic farming practices. Hence, it is evident that these microbes can favorably be used to restore soil health and productivity and promote plant growth. Nevertheless, there is a need to carry out long-term studies to unfold their long-term sustainability and to further understand the concept of interactions between microbes and plants in the soil if a more effective reclamation of degraded soils is to be achieved.
Different endophytic and rhizospheric bacteria have distinctive strategies for enhancing plant growth and yield in the soil. The distinct biotic activities of these bacteria make them vital components for nutrient restoration in sustainable farming systems [63] (Figure 3). They produce extracellular fluids such as organic acids that solubilize nutrients for easy absorption by plants [64]. Other endophytic bacteria indirectly promote plant growth through biocontrol mechanisms such as competition for nutrients, release of lytic enzymes, and the induction of plant defense mechanisms [13]. This is supported by the findings of Devi et al. [65], where the application of Bacillus spp. induced systemic resistance and nutrient competitive advantage in inoculated potato plants. The inter-specific plant-microbial interaction process starts with the establishment of communication between the host plant and microorganism. This is made possible by the production of specific compounds in the root exudates that aid signaling and recognition. The type of root exudates significantly determines the composition of microbiota in the rhizosphere colonizing the plant [66]. Through a series of complex signal mediated communications, bacterial endophytes can gain entry into the root's endosphere such as nodules in the case of legumes.
Apart from water and temperature, plants in terrestrial environments are strongly constrained by nutrients availability. Notably, plants in different ecosystems respond to changes in the concentration of one or more soil nutrients and endophytes can play a key role in balancing soil nutrients, enhancing nutrient acquisition, and plant growth [67]. In degraded soil, for instance, there is low availability of minerals such as P and Fe for plant absorption. Acquisition of such nutrients can be enhanced by the use of bacterial endophytes that releases phytosiderophores responsible for Fe chelation and carboxylate exudates required for P mobilization [68,69].
Endophytic bacteria can perform various nutrient transformations, thanks to their versatile enzymatic systems crucial in nutrient recycling and metabolism [70]. The transformation of atmospheric N2 through biological nitrogen fixation (BNF) is important to the plants and in restoring soil fertility. BNF is a microbial mediated process that involves converting atmospheric N2 in the presence of nitrogenase enzyme complex into ammonia and nitrate [71]. Nitrogen is considered a major growth-limiting nutrient in plants. The microbes involved in this kind of nutrient conversion are referred to as diazotrophs. According to Nag et al. [72], some diazotrophs can fix nitrogen in association with their host plants, while others can do so in their free-living state. Some symbiotic bacterial endophytes induce physiological and structural modifications of plant roots leading to the establishment of specialized structures known as nodules. For instance, legume plants usually establish specific associations with soil rhizobacteria that can sequester N2 as ammonia using root nodules. These include Bradyrhizobium, Rhizobium, Allorhizobium, Sinorhizobium, and Mesorhizobium [73,74,75]. Other soil microorganisms such as actinomycetes and cyanobacteria in cycads and lichens also have been reported to fix nitrogen through the formation of symbiotic associations [76].
Symbiotic associations tend to work efficiently because the N fixed by the bacteria is directly transferred to the host plant and in return, the photosynthetically fixed carbon and other metabolites benefit the bacterial symbiont [70]. However, there are nitrogen-fixing bacteria that are non-symbionts and do not induce nodulation unlike rhizobia (Figure 4). These bacteria have the ability to thrive on the nutrients and energy derived from the roots of the host plant. According to Afzal et al. [22] and Njeru et al. [77], associative nitrogen-fixing endophytes can promote plant growth and health in nitrogen-limited soils compared to other rhizospheric microorganisms. Similarly, endophytic bacteria that are nitrogen fixers can enhance nitrogen accumulation in plants growing in nitrogen-deficient soils as described by Gupta et al. [78]. The success of culturable N-fixing endophytes in promoting plant growth in poor field conditions indicates the reliability of the endophytes in delivering low-cost ecosystem services for farmers. However, it is important to note that there are less endophytic bacteria that have enzymatic ability to carry out BNF compared to the total endophytic bacterial population. Hence, more studies should be carried out to identify candidate endophytes that thrive in stressful environments and have high N-fixing potential with legumes and non-legumes.
Plants require phosphorus (P) as an essential nutrient for growth and development. The deficiency of P availability in the rhizosphere is a major limiting factor for plant growth. P is involved in important plant-metabolic processes such as respiration, biosynthesis of macromolecules, energy transfer, and photosynthesis [79]. Phosphorus in the soil is often in forms that are unavailable for plant uptake and hence cannot support plant growth. According to Alori et al. [80], about 95% of phosphate in the soil is immobilized, insoluble, and/or precipitated into minerals like rock phosphate and tricalcium phosphate. Interestingly, phosphorus applied using inorganic fertilizers end up forming complexes with the soil and hence becoming unavailable for plant absorption [81]. To increase P availability in the soil, mineralization and solubilization of phosphate through biological processes are required. These processes are carried out effectively by phosphate solubilizing bacteria, which are part of endophytic bacteria colonizing the plants and rhizosphere [80]. Among the most effective groups of solubilizers include Pseudomonas, Enterobacter, Bacillus, Mesorhizobium, Rhizobium, Achromobacter, and Acinetobacter [36,82].
Endophytic bacteria promote P availability by solubilizing the insoluble P forms using mechanisms such as organic acid production, acidification, ion exchange and chelation [82]. Other endophytic bacteria enhance P solubilization by secretion of complexes in the soil that mineralize organic phosphorus [83]. For instance, Burkholderia sp. synthesizes tartaric, citric and oxalic acids that are largely involved in phosphorus solubilization [84]. During vermicomposting, inoculation of plants using Herbaspirillum seropedicae, Bacillus spp., and Burkholderia silvatlantica increases P availability and phosphatase activity [85]. Besides, bacterial endophytes can assimilate solubilized P, hinder soil P fixation, and promote adsorption of phosphate into the host plant under phosphate limiting conditions, thus, making it more available for the plants [86]. Therefore, endophytic bacteria can be used as potential biofertilizers for restoring soil fertility in degraded soils. They are part of the most promising sustainable interventions in agriculture due to their effectiveness and environmentally friendly nature. Because of their efficient interventions in P solubilization, endophytes remain the most viable biological resource systems that have been identified to date. They have been proven to solubilize inorganic P into available forms such as orthophosphate improving plant growth and yield in degraded soils [80].
Potassium (K) not only provides resilience to both abiotic and biotic stresses, but also plays a key role in plant metabolic and physiological processes. Unfortunately, over 90% of K in the soil is unavailable for plant absorption necessitating to search for effective endophytic bacteria and rhizobacteria that solubilize the crucial mineral. The limited availability of K in the soil is further depleted by intensive farming, especially in developing countries where farmers do not put adequate strategies to replenish the lost soil K nutrient after a harvest [87]. According to Minden and OldeVenterink [88], K deficiency worldwide is considered a major setback in crop production. The fact that farmers apply inorganic fertilizers without knowing the exact standard amount required worsens the situation. Continuous use of chemical fertilizers is environmentally undesirable and a costly affair in maintaining soil fertility status. Therefore, to sustain crop production, it is important to improve K availability by finding alternative sustainable ways.
It has been demonstrated that some endophytic bacteria establish a functional relationship with plants that is holistic in nature and contributes to K bioavailability [13]. Endophytic bacteria can free potassium from insoluble soil minerals and they are referred to as K-solubilizing bacteria (KSB). A large diversity of bacterial endophytes that can also carry out K solubilization includes; Burkholderia spp. Acidithiobacillus ferrooxidans, Flavobacterium spp. Bacillus circulans, Bacillus mucilaginosus, Paenibacillus spp. and Bacillus edaphicus [89]. The B. mucilaginosus and B. edaphicus have demonstrated high capabilities in K solubilization from K-bearing minerals [90,91]. These microbes are known to utilize mechanisms such as chelation, production of organic acids, complexation, acidolysis, exchange reactions and lowering soil pH to cause the dissolution of K in the soils [92]. Several studies have shown that KSBs, under controlled and field conditions, can improve crops' germination, growth, uptake of nutrients, and yields [91,93].
The use of endophytic and rhizospheric bacteria in K solubilization may not entirely fulfill the K requirements for the plants as compared to the use of inorganic K fertilizers [28]. However, K availability can be enhanced significantly by the novel approach of using rhizobacteria which are eco-friendly and can greatly cut the use of inorganic fertilizers in crop production [93]. For instance, some K solubilizing bacteria that have been used to improve agricultural soils and productivity of specific crops include Pseudomonas and Bacillus spp. in sorghum (Sorghum bicolor), Pseudomonas spp., Bacillus spp., Micrococcus spp. and Enterobacter spp. in common beans (Phaseolus vulgaris), Mesorhizobium ciceri and P. jessenii in chickpea (Cicer arietinum) [94], Bacillus, Paenibacillus kribbensis, Stenotrophomonas and Pseudomonas in wheat (Triticum aestivum) [95], Bacillus circulans in Oranges (Citrus sinensis), and Bacillus spp., Azotobacter spp., Pseudomonas spp. and Mesorhizobium spp. in various legumes [96,97,98].
The use of endophytic bacteria in the restoration of K degraded soils is considered an attractive and pragmatic intervention for sustainable crop production. According to Etesami et al. [99], K bacterial solubilizers are valued resources for lessening K deficiencies in degraded agricultural soils. However, there is still grossly inadequate experimental evidence on their effectiveness in degraded field conditions. Additionally, studies by Meena et al. [91] shows that the approach is not well utilized due to lack of sufficient information and awareness in communities practicing agriculture for their livelihood.
The productivity of crops is threatened by the increasing biotic and abiotic stresses across the globe, of which some are linked to the effects of climate change while others are anthropogenic [100]. The incidences of extreme events like the emergence of plant diseases and pests, frost, heat waves, intense rains coupled with floods, and severe drought are increasingly being witnessed globally [101]. These incidences are expected to continue increasing due to the changing climate and their impacts are thought to severely affect the livelihoods of many people in developing countries. Plant breeding has been utilized to produce genotypes that can tolerate stresses but their success in the field has been limited and is not guaranteed to hold the changing future climatic conditions [102]. In this context, soil microorganisms have unique traits that can reduce the severity of the incidences and promote sustainable crop production. The endophytic bacteria and rhizobacteria have shown a high capability of enhancing crop growth and yield by inducing tolerance traits against different forms of stress [103]. These microbes exhibit multifaceted functional traits and can colonize and firmly establish themselves in the plant tissues, thereby positively influencing plant growth and survival. Studies have shown that endophytic bacteria can increase the supply of nutrients to their host plants to enable them to manage stress and suppress plant nematodes and insect pests [104]. Besides, endophytic bacteria can reduce disease severity and suppress weed growth, thus, improving plant resilience under stress conditions [105].
According to Gorai et al. [106] plants with high endophytic and rhizospheric microbial diversity are more resilient to environmental stress as opposed to endophytic-free plants. Additionally, Vigani et al. [107] suggested that endophytic bacteria can sustain their host plants against different forms of stress by developing resistance "power". Endophytic microbes synthesize various secondary metabolites or antistress-like metabolites that activate the host plant's stress management mechanisms. Some of those metabolites include phytohormones (ethylene and abscisic acid), enzymes (superoxide dismutase and catalase), and organic osmolytes such as glycine, butane and proline [108]. Some endophytes induce the production of reactive oxygen as scavenger species that deal with free radicals, while others trigger antagonistic actions and defensive pathways to suppress phytopathogens [109]. However, Le Cocq et al. [20] warn of possible toxin production by endophytes while suppressing plant stress and hence it would be imperative to rigorously test different metabolites that they produce for animal and human safety.
Endophytic bacteria and rhizobacteria also confer stress resilience via induction of plant immune fitness, and biocontrol of destructive insects, pests and phytopathogens. Biological control is described as a mechanism of protecting plants against phytopathogens through the production of bacteriocins, lytic enzymes, antibiotics and siderophores. Endophytic inoculation has been shown to suppresses fungal, bacterial, and viral diseases [110]. Bacillus amyloliquefaciens have shown high potential as a biocontrol agent against powdery mildew in tobacco plants [111]. Similarly, verticillium wilt disease in cotton that is caused by Verticillium dahliae has been suppressed significantly by the application of Enterobacter spp. in pot and field experiments [112]. According to Beneduzi et al. [113], rhizosphere and endophytic bacteria suppress diseases by inducing systemic resistance, which is a defensive mechanism developed by plants after stimulation.
The use of bacterial endophytes that produce antibiotics in the host makes them very efficient in combating plant pathogens [114]. Examples of antibiotic compounds are lipopeptides biosurfactants that are synthesized by Bacillus spp. and Pseudomonas spp. According to Wang et al. [115], antibiotic compounds isolated from different bacterial endophytes affect other organisms by inhibiting cell wall synthesis and ribosomes' small subunit formation [115]. Azospirillum spp. produces plant metabolites and hormones such as auxins in retaliation to stress conditions like carbon limitation, drought stress [19] and high acidity and salinity [116]. Endophytic bacteria and other rhizobacteria can mitigate environmentally induced stresses and enhance plant survival under challenging environmental conditions. These roles are expected to be more relevant with the increasingly adverse effects of climate change on soil quality, health and food production [117]. However, the activity and effectiveness of endophytes depends on plant species, growth stage, age, and other plant genotypic properties, and most importantly the changing environmental conditions [118].
The application of biofertilizers from microbe-based products has been demonstrated in a burgeoning volume of literature [119]. For instance, for many decades rhizobial biofertilizers have been utilized commercially reducing the need for inorganic chemical fertilizer application. Many companies and organizations in different countries have also participated or engaged in the production of biofertilizers commercially [120]. However, the maximum utilization of beneficial endophytic and rhizospheric bacteria in crop production remains largely unexplored [22]. This may be driven by a lack of market penetration for biofertilizers, especially smallholder farmers who need the technology most. Farmers are not aware of a wide range of potential endophytes apart from the few commonly used species such as Rhizobium and Bacillus spp. and this impedes their continuous use and adoption [121]. Despite the many roles played by the PGPRs and bacterial endophytes in promoting plant growth, several setbacks such as tough competition and harsh environmental conditions still limit their effectiveness under degraded field conditions [122]. Lesueur et al. [122] highlights that despite the availability of a vast number of commercial bioinoculants, the efficiency and quality of the majority are not proven. In our view, the overdependence of inorganic fertilizers can only be reduced by the presence of consistent and good efficacious microbial bioinoculants [123].
Certainly, the use of beneficial soil microorganisms could be convenient for the farmers because of their resilient nature in the environment. Bacteria species such as Bacillus are gram-positive and can survive detrimental conditions by producing endospores [124]. The endospores allow the microbes to withstand different seasons and environmental variables, thereby supporting plant growth in changing climatic conditions. According to Moawad et al. [125], microbial inoculants' persistence varies from one strain to another. Some microbial inoculants such as Rhizobium etli, Bradyrhizobium japonicum and Rhizobium phaseoli can persist in the environment and plant materials for years although ineffective due to low abundance. Contrary, some microbial inoculants decrease below detectable levels within weeks like the cases of Bacillus amyloliquefaciens and Trichoderma harzianum [126].
It is important to consider transferring useful soil microbes from laboratory to farms in the field. The transfer of this technology to the field involves testing and making the best choice for microbial strain, mass production and appropriate handling including having the right carrier material to ensure the microbes remain viable for a given period [127]. Additionally, quality control is important to monitor the preparation process, packaging and storage conditions. For instance, microbial inoculants packaged in polybags with a carrier material such as soil and dairy animal waste powder can be stored for 3–4 months at 28 ± 2 ℃ [128]. In most cases during development, there is no consideration of the burden that the microbial inoculants have to withstand and overcome the harsh and competitively agammaessive soil environment [82]. Therefore, the inoculated endophytes must contend with the local microflora and adjust to the flighty and heterogeneous soil environment. In addition, efficient quality control should be done to ensure the availability of excellent and reliable inoculants for farmers. According to Parnell et al. [129], adoption of this technology by farmers is also another hurdle because they are unpopular which may be due to a lack of awareness. The majority of the farmers in developing countries are smallholders and based on their economic situation, they expect instant results from endophyte bioinoculation. This may not happen immediately depending on the nature of the soils, application method and crop genotype cultivated. The situation may be further worsened by the poorly-developed biofertilizer supply chain [130].
The use of endophytic and rhizospheric bacteria is a viable approach that is recommended for use by farmers. There exists various evidence for the success of these microbial inoculants. For example, there was a significant increase in plant height, pod and leaf/stem biomass of Brassica napus crop after inoculation with Pseudomonas fluorescens and a bacterial consortium in both field and greenhouse conditions [131]. According to Alkahtani et al. [132], endophytic bacteria are good PGPR candidates for use to increase stress resilience and nutrient uptake in plants while reducing chemical inputs used in conventional farming. In the same study, Brevibacillus and Bacillus strains were identified as the most common endophytes and had a significant increase in shoot N and P content. Similarly, co-inoculation of endophytic and rhizospheric bacteria in Roshan and Marvdasht wheat cultivars enhanced plant shoot dry weight (by 5.8% and 7.5%) and height (by 15.0% and 11.0%) respectively in P deficient soils while P utilization efficiency increased by 29.5% and 18.7% for Marvdasht and Roshan wheat cultivars respectively [133]. Interestingly, the same study showed that co-inoculation of endophytic and rhizospheric bacteria act synergistically in improving plant growth and P acquisition. Despite the many successful greenhouse or pot trials reported on the use of endophytes, their long-term effects in supporting plant growth in degraded fields remain largely unaccounted. Studies have demonstrated the usefulness of microbial inoculants in crop production as summarized in Table 1 and Table 2.
Crop | Endophytic bacteria | Bioactive activity | References |
Maize (Zea mays) | Enterobacter spp. | Disease suppression (Against Fusarium verticillioides) Seedling growth and health | [134] |
Burkholderia spp. | Enhance growth and P-utilization | [135] | |
Wheat | Enterobactereacea (Pantoea genus) | IAA and siderophore producer, and also solubilised phosphate biocontrol abilities against Fusarium graminearum | [136] |
Banana (Musa spp.) | Enterobacter cloacae | Promote growth and health | [137] |
Finger millet (Eleusine coracona (L). Gaertner) | Pseudomonas spp. | Blast disease management and growth promotion | [138] |
Oryza sativa (rice), Arachis hypogaea (groundnut), Vigna mungo (black gram) | Enterobacter cloacae | Enhance seed germination index, shoot and root biomass of seedling, seed vigour index and salinity tolerance | [139,140] |
Peanut (Arachis hypogaea L.) | Bradyrhizobium and Trichoderma | Growth improvement | [141] |
Groundnut (Arachis hypogaea L.) | Bacillus subtilis | Suppression of stem rot caused by Sclerotium rolfsii and growth promotion | [142] |
Cowpea (Vigna unguiculata) | Pseudomonas fluorescens | Seed health and yield | [143] |
Damping off control caused by Sclerotium rolfsii | |||
Bacillus pumilus and Bacillus subtilis | Resistance against the black eye cowpea mosaic strain | [144] | |
Common Bean (Phaseolus vulgaris L.) | Trichoderma atroviridae | Biocontrol against common bean root rot (Fusarium solani) and growth enhancement. | [145] |
Rhizobium | Nitrogen (N) fixation | ||
Bacillus spp. | Biocontrol activity of Fusarium sp., Macrophomina sp., and Alternaria sp | [146] | |
Bacillus amyloliquefaciens | Biocontrol against charcoal root rot | [147] | |
Soybean (Glycine max) | Bradyrhizobium | Nitrogen fixation | [148] |
Bacillus cereus | Mitigation of heat stress damage | [149] | |
Bacillus firmus (SW5) | Enhancing salt tolerance | [150] | |
Pseudomonas koreensis | Salt sress tolerance in soybean | ||
Pseudomonas pseudoalcaligenes | Alleviates salt stress in soybean plants | [151] | |
Bacillus spp. | Biological control of the rootknot nematode, Meloidogyne javanica (Chitwood) | [152] | |
Cassava | Bacillus amyloliquefaciens and Microbacterium imperiale | Mitigates Fusarium root rot disease | [153] |
African cultivated rice (Oryza glaberrima) | Photosynthetic Bradyrhizobium | Increase in the shoot growth and grain yield | [154] |
Tomato | Stenotrophomonas sp. str. S33 (KR818084) and Pseudomonas sp | Suppress tomato Fusarium wilt disease caused by Fusarium oxysporum f. sp. Lycopersici (FOL) | [155] |
Bacillus amyloliquefaciens | Drought tolerance | [156] | |
Bacillus cereus | Thermotolerance | [157] | |
Rice (Oryza sativa) | Bacillus subtilis | Disease suppression against fungal pathogens Rhizoctonia solani, Fusarium verticelloides and Sclerotium rolfsii.antibacterial activities against Xanthomonas oryzae | [158] |
Sugracane (Saccharum officinarum) | Gluconacetobacter diazotrophicus | Drought tolerance | [159] |
Date palm tree (Phoenix dactylifera L.) | Paenibacillus xylanexedens) and (Enterobacter cloacae) | Facilitate nutrient uptake in roots enhance canola root elongation | [140] |
Sweet potato (Ipomoea batatas (L.) Lam.) | Bacillus amyloliquefaciens | Resistance against fungal pathogens | [160] |
Sunflower (Helianthus annuus L.) | Burkholderia | Stimulate plant growth | [161] |
Sorghum (Sorghum bicolor) | Gluconacetobacter diazotrophicus | Nitrogen fixation | [162] |
Burkholderia tropica | Growth promotion | [163] |
Rhizobacteria | Growth promotion traits | Associated rhizosphere | References |
Stenotrophomonas spp | Enhance nutrients uptake and growth | Wheat (Triticum aestivum L.) | [164] |
Chryseobacterium antibioticum | Antimicrobial activity against gram-negative bacteria.Siderophore production | Arctic soil | [165] |
Gluconacetobacter diazotrophicus | Drought tolerance Nitrogen fixation | Sugarcane (Saccharum officinarum) | [159] |
Paenibacillus mucilaginosus | IAA production Potassium solubilization | Apple (Malus domestica) | [166] |
Phyllobacterium ifriqiyense and Phyllobacterium sophorae | Siderophore production Phosphate solubilization | Wheat (Triticum aestivum L.) | [167] |
Pseudomonas aeruginosa | Biocontrol agents against bacterial blight disease Stress management through glucanase and chitinase production | Rice (Oryza sativa) | [168] |
Bacillus velezensis | Biocontrol agent against corynespora leaf spot diseases | Cucumber (Cucumis sativus) | [169] |
Pseudomonas sp | Salinity tolerance | Sunflower (Helianthus annuus) | [170] |
Azospirillum | Biofertilizer | Barley (Hordeum vulgare), wheat (Triticum aestivum L.), oats | [171] |
Bacillus cereus | Phytoremediation of heavy metals | Vetiveria zizanioides L | [172] |
Bacillus spp. | Auxin synthesis Production of antibiotics, siderophores and enzymes | Potato (Solanum tuberosum), cucumber (Cucumis sativus), peanuts (Arachis hypogaea) | [173] |
Bacillus amyloliquefaciens | Mitigation of nitrous oxide emissions | Acidic soils | [174] |
Burkholderia kururiensis | Nutrients uptake Nitrogen fixation | Sorghum (Sorghum bicolor) | [175] |
Nitrosospira spp. | Management and control of nitrous oxide in soils with excess use of inorganic fertilizers | Tropical soils | [176] |
Pseudomonas putida | Iron translocation through siderophore production | Mung bean (Vigna radiata) | [177] |
Pseudomonas fluorescens | Biocontrol agent against fusarium wilt disease | Tomato (Solanum lycopersicum) | [178] |
Burkholderia paludis | Siderophore and antibiotic production | Forest rhizosphere | [179] |
Bacillus cepacia | Biocontrol agent against fungal pathogens | Pepper plant (Piper nigrum) | [180] |
Rhodobacteria | Nitrogen fixation | Wheat (Triticum aestivum L.) | [181] |
Azarcus | Nitrogen fixation | Rice (Oryza sativa) | [182] |
Azorhizobium | Nitrogen fixation | Sugarcane (Saccharum officinarum) | [183] |
SerratiaMarcescens | Pathogen biocontrol Antifungal | Wheat (Triticum aestivum L.) | [184] |
Pseudomonas aeruginosa | Siderophore production Biocontrol agent against Rhizoctonia solani and Colletotrichum gloeosporioides | Chilli (Capsicum frutescens) | [185] |
To develop and increase the use of effective bacterial endophytes in modern crop production, it would be important to expand the endophyte screening and the understanding of their physiological functioning using state-of-the-art technologies such as high-throughput sequencing, meta-transcriptomics and metagenomics. Broadening the understanding of the full genome of endophytic bacteria, aiming to uncover the functional genes that help them adapt to unfavorable conditions, would be a breakthrough in biotechnology and agriculture. For instance, the application of fertilizers in crop production could be minimized or avoided by the identification of highly effective endophytes that gain entry and colonize the plant tissues and overcome the plant immune response and infer resilience to abiotic and biotic stressors including the changing climatic conditions. Further in-depth research would be crucial to maximizing the benefits of endophytic bacteria in sustainable agriculture through a better understanding of their ecological roles and associations. The practical association of bacterial endophytes with plants in the field can be explored for the potential restoration of degraded and unproductive lands.
Bacterial endophytes play a very crucial role in enhancing and promoting plant growth and resilience against abiotic and biotic stress. These bacteria promote plant growth by actively enhancing nutrient availability, biomass production, leaf area, hydraulic activity, chlorophyll content, shoot and root ratio, and tolerance against acidity, drought among other forms of stress. Studies from different bioassays carried out in both field and microcosms conditions have shown that bacterial endophytes and rhizobacteria are potential plant probiotics championed to enhance nutrients bioavailability in the soil. In this regard, the successful use of endophytic bacteria can reduce significantly the indiscriminate use of artificial chemicals such as inorganic fertilizers and pesticides although this change may not take place drastically without compromising other norms valued in conventional farming. The endophytic and rhizospheric bacterial communities have shown the potential to enhance the bioavailability of natural nutrients that are often limited for soil enrichment and better crop production. There is an imperative call for more information on the fate of microbial inoculants in degraded soils and their interaction with plants and indigenous microbes. There is a growing need to improve nutrients use efficiency in the soil in an eco-friendly manner, which can be achieved by the application of microbial nutrients solubilizers. This kind of information will certainly pave way for the adoption of bioinoculants in crop productivity based on their potential in actual field conditions.
SMW provided the outline structure and prepared the manuscript in collaboration with GK. EMN and JMM provided guidance, feedback and technical review during manuscript development. All authors contributed to editing, revising and approving the final draft of the manuscript submitted.
This work was supported by The Future Leaders – African Independent Researchers (FLAIR) Fellowship Programme, which is a partnership between the African Academy of Sciences and the Royal Society funded by the UK Government's Global Challenges Research Fund (Grant number FLR\R1\190944). The Scuola Superiore Sant'Anna, Pisa, Italy and the Deutscher Akademischer Austauschdienst (DAAD) funded the PhD scholarships of G. Koskey and S. Mburu respectively.
Authors declare there is no conflict of interest.
The authors acknowledge the Chuka University, Kenya and the Kenyatta University FLAIR research team (Nairobi) led by Dr. E.M. Njeru for their collaboration and in provision of supporting materials included in this manuscript.
[1] | Vanlauwe B, Descheemaeker K, Giller KE, et al. (2014) Integrated soil fertility management in sub-Saharan Africa. Soil Discuss 1: 1239-1286. |
[2] |
Kopittke PM, Menzies NW, Wang P, et al. (2019) Soil and the intensification of agriculture for global food security. Environ Int 132: 105078. doi: 10.1016/j.envint.2019.105078
![]() |
[3] | Singh A, Kumar M, Verma S, et al. (2020) Plant microbiome: Trends and prospects for sustainable agriculture. In: Varma A, Tripathi S, Prasad R. (Eds), Plant microbe symbiosis. Springer, Cham, 129-151. |
[4] | Altieri MA, Farrell JG, Hecht SB, et al. (2018) The Agroecosystem: Determinants, resources, processes, and sustainability. Agroecology. CRC Press, 41-68. |
[5] | Kumar J, Singh D, Ghosh P, et al. (2017) Endophytic and epiphytic modes of microbial interactions and benefits. Plant-microbe interactions in agro-ecological perspectives. Singapore: Springer, 255-771. |
[6] | Ali MA, Naveed M, Mustafa A, et al. (2017) The good, the bad, and the ugly of rhizosphere microbiome. In: Kumar V, Kumar M, Sharma S, et al. (Eds), Probiotics and plant health. Singapore: Springer, 253-290. |
[7] |
Kumar A, Verma JP (2018) Does plant-Microbe interaction confer stress tolerance in plants: A review? Microbiol Res 207: 41-52. doi: 10.1016/j.micres.2017.11.004
![]() |
[8] | Glick BR (2020) Introduction to plant growth-promoting bacteria. beneficial Plant-Bacterial Interactions. Springer, Cham, 1-37. |
[9] | Verma M, Mishra J, Arora NK (2019) Plant growth-promoting rhizobacteria: Diversity and applications. In: Sobti R, Arora N, Kothari R. (Eds), Environmental Biotechnology: For Sustainable Future. Singapore: Springer, 129-173. |
[10] |
Bhattacharyya PN, Jha DK (2012) Plant growth-promoting rhizobacteria (PGPR): Emergence in agriculture. World J Microbiol Biotechnol 28: 1327-1350. doi: 10.1007/s11274-011-0979-9
![]() |
[11] |
Pii Y, Mimmo T, Tomasi N, et al. (2015) Microbial interactions in the rhizosphere: beneficial influences of plant growth-promoting rhizobacteria on nutrient acquisition process. A review. Biol Fertil Soils 51: 403-415. doi: 10.1007/s00374-015-0996-1
![]() |
[12] |
Prasad M, Srinivasan R, Chaudhary M, et al. (2019) Plant growth promoting rhizobacteria (PGPR) for sustainable agriculture. PGPR Amelior Sustain Agric 129-157. doi: 10.1016/B978-0-12-815879-1.00007-0
![]() |
[13] |
Liu HW, Carvalhais LC, Crawford M, et al. (2017) Inner plant values: Diversity, colonization and benefits from endophytic bacteria. Front Microbiol 8: 2552. doi: 10.3389/fmicb.2017.02552
![]() |
[14] |
Eljounaidi K, Lee SK, Bae HH (2016) Bacterial endophytes as potential biocontrol agents of vascular wilt diseases-Review and future prospects. Biol Control 103: 62-68. doi: 10.1016/j.biocontrol.2016.07.013
![]() |
[15] |
Ullah A, Nisar M, Ali H, et al. (2019) Drought tolerance improvement in plants: an endophytic bacterial approach. Appl Microbiol Biotechnol 103: 7385-7397. doi: 10.1007/s00253-019-10045-4
![]() |
[16] | Pandey PK, Samanta R, Yadav RNS (2019) Inside the plant: addressing bacterial endophytes in biotic stress alleviation. Arch Microbiol 201: 415-429. |
[17] |
Rabiey M, Hailey LE, Roy SR, et al. (2019) Endophytes vs tree pathogens and pests: can they be used as biological control agents to improve tree health? Eur J Plant Pathol 155: 711-729. doi: 10.1007/s10658-019-01814-y
![]() |
[18] |
Griffiths SM, Galambao M, Rowntree J, et al. (2020) Complex associations between cross-kingdom microbial endophytes and host genotype in ash dieback disease dynamics. J Ecol 108: 291-309. doi: 10.1111/1365-2745.13302
![]() |
[19] |
zhang Y, Yu XX, Zhang WJ, et al. (2019) Interactions between endophytes and plants: Beneficial effect of endophytes to ameliorate biotic and abiotic stresses in plants. J Plant Biol 62: 1-13. doi: 10.1007/s12374-018-0274-5
![]() |
[20] |
Le Cocq K, Gurr SJ, Hirsch PR, et al. (2017) Exploitation of endophytes for sustainable agricultural intensification. Mol Plant Pathol 18: 469-473. doi: 10.1111/mpp.12483
![]() |
[21] | Singh M, Kumar A, Singh R, et al. (2017) Endophytic bacteria: a new source of bioactive compounds. 3 Biotech 7: 316. |
[22] |
Afzal I, Shinwari ZK, Sikandar S, et al. (2019) Plant beneficial endophytic bacteria: Mechanisms, diversity, host range and genetic determinants. Microbiol Res 221: 36-49. doi: 10.1016/j.micres.2019.02.001
![]() |
[23] | Velázquez E, Carro L, Flores-Félix JD, et al. (2017) The legume nodule microbiome: A source of plant growth-promoting bacteria. In: Kumar V, Kumar M, Sharma S, et al. (Eds), Probiotics and plant health. Singapore: Springer, 41-70. |
[24] |
Leite J, Fischer D, Rouws LFM, et al. (2017) Cowpea nodules harbor non-rhizobial bacterial communities that are shaped by soil type rather than plant genotype. Front Plant Sci 7: 2064. doi: 10.3389/fpls.2016.02064
![]() |
[25] |
Sharaf H, Rodrigues RR, Moon JY, et al. (2019) Unprecedented bacterial community richness in soybean nodules vary with cultivar and water status. Microbiome 7: 63. doi: 10.1186/s40168-019-0676-8
![]() |
[26] |
Compant S, Samad A, Faist H, et al. (2019) A review on the plant microbiome: Ecology, functions, and emerging trends in microbial application. J Adv Res 19: 29-37. doi: 10.1016/j.jare.2019.03.004
![]() |
[27] |
Orozco-Mosqueda M del C, Rocha-Granados M del C, Glick BR, et al. (2018) Microbiome engineering to improve biocontrol and plant growth-promoting mechanisms. Microbiol Res 208: 25-31. doi: 10.1016/j.micres.2018.01.005
![]() |
[28] |
Kour D, Rana KL, Yadav AN, et al. (2020) Microbial biofertilizers: Bioresources and eco-friendly technologies for agricultural and environmental sustainability. Biocatal Agric Biotechnol 23: 101487. doi: 10.1016/j.bcab.2019.101487
![]() |
[29] |
Thiergart T, Durán P, Ellis T, et al. (2020) Root microbiota assembly and adaptive differentiation among European Arabidopsis populations. Nat Ecol Evol 4: 122-131. doi: 10.1038/s41559-019-1063-3
![]() |
[30] |
Thomas P, Sekhar AC (2017) Cultivation versus molecular analysis of banana (Musa sp.) Shoot-tip tissue reveals enormous diversity of normally uncultivable endophytic bacteria. Microb Ecol 73: 885-899. doi: 10.1007/s00248-016-0877-7
![]() |
[31] |
Goulart MC, Cueva-Yesquén LG, Martinez KJH, et al. (2019) Comparison of specific endophytic bacterial communities in different developmental stages of Passiflora incarnata using culture-dependent and culture-independent analysis. Microbiologyopen 8: e896. doi: 10.1002/mbo3.896
![]() |
[32] |
Fadiji AE, Babalola OO (2020) Metagenomics methods for the study of plant-associated microbial communities: A review. J Microbiol Meth 170: 105860. doi: 10.1016/j.mimet.2020.105860
![]() |
[33] |
Sarhan MS, Hamza MA, Youssef HH, et al. (2019) Culturomics of the plant prokaryotic microbiome and the dawn of plant-based culture media-A review. J Adv Res 19: 15-27. doi: 10.1016/j.jare.2019.04.002
![]() |
[34] |
Beckers B, Op De Beeck M, Thijs S, et al. (2016) Performance of 16s rDNA primer pairs in the study of rhizosphere and endosphere bacterial microbiomes in metabarcoding studies. Front Microbiol 7: 650. doi: 10.3389/fmicb.2016.00650
![]() |
[35] |
Kunda P, Dhal PK, Mukherjee A (2018) Endophytic bacterial community of rice (Oryza sativa L.) from coastal saline zone of West Bengal: 16S rRNA gene based metagenomics approach. Meta Gene 18: 79-86. doi: 10.1016/j.mgene.2018.08.004
![]() |
[36] | Suman A, Yadav AN, Verma P (2016) Endophytic microbes in crops: Diversity and beneficial impact for sustainable agriculture. In: Singh D, Singh H, Prabha R. (Eds), Microbial inoculants in sustainable agricultural productivity. New Delhi: Springer, 1: 117-143. |
[37] |
Cheng DD, Tian ZS, Feng L, et al. (2019) Diversity analysis of the rhizospheric and endophytic bacterial communities of Senecio vulgaris L. (Asteraceae) in an invasive range. PeerJ 6: e6162. doi: 10.7717/peerj.6162
![]() |
[38] | Ferrando L, Fernández-Scavino A (2013) Functional diversity of endophytic bacteria. In: Aroca R. (Ed), Symbiotic Endophytes. Heidelberg: Springer, 195-211. |
[39] | Kour D, Rana KL, Yadav N, et al. (2019) Rhizospheric microbiomes: Biodiversity, mechanisms of plant growth promotion, and biotechnological applications for sustainable agriculture. In: Kumar A, Meena V. (Eds), Plant growth promoting rhizobacteria for agricultural sustainability. Singapore: Springer, 19-65. |
[40] |
Liotti RG, da Silva Figueiredo MI, da Silva GF, et al. (2018) Diversity of cultivable bacterial endophytes in Paullinia cupana and their potential for plant growth promotion and phytopathogen control. Microbiol Res 207: 8-18. doi: 10.1016/j.micres.2017.10.011
![]() |
[41] |
Harrison JG, Griffin EA (2020) The diversity and distribution of endophytes across biomes, plant phylogeny and host tissues: how far have we come and where do we go from here? Environ Microbiol 22: 2107-2123. doi: 10.1111/1462-2920.14968
![]() |
[42] |
Jha CK, Patel D, Rajendran N, et al. (2010) Combinatorial assessment on dominance and informative diversity of PGPR from rhizosphere of Jatropha curcas L. J Basic Microbiol 50: 211-217. doi: 10.1002/jobm.200900272
![]() |
[43] |
Andreolli M, Lampis S, Zapparoli G, et al. (2016) Diversity of bacterial endophytes in 3 and 15 year-old grapevines of Vitis vinifera cv. Corvina and their potential for plant growth promotion and phytopathogen control. Microbiol Res 183: 42-52. doi: 10.1016/j.micres.2015.11.009
![]() |
[44] |
Koskey G, Mburu SW, Kimiti JM, et al. (2018) Genetic characterization and diversity of Rhizobium isolated from root nodules of mid-altitude climbing bean (Phaseolus vulgaris L.) Varieties. Front Microbiol 9: 968. doi: 10.3389/fmicb.2018.00968
![]() |
[45] |
Wang L, Cao Y, Wang ET, et al. (2016) Biodiversity and biogeography of rhizobia associated with common bean (Phaseolus vulgaris L.) in Shaanxi Province. Syst. Appl. Microbiol 39: 211-219. doi: 10.1016/j.syapm.2016.02.001
![]() |
[46] |
Chakraborty P, Tribedi P (2019) Functional diversity performs a key role in the isolation of nitrogen-fixing and phosphate-solubilizing bacteria from soil. Folia Microbiol (Praha) 64: 461-470. doi: 10.1007/s12223-018-00672-1
![]() |
[47] |
Taylor BN, Simms EL, Komatsu KJ (2020) More than a functional group: Diversity within the legume-rhizobia mutualism and its relationship with ecosystem function. Diversity 12: 50. doi: 10.3390/d12020050
![]() |
[48] |
Prasannakumar MK, Mahesh HB, Desai RU, et al. (2020) Metagenome sequencing of fingermillet-associated microbial consortia provides insights into structural and functional diversity of endophytes. 3 Biotech 10: 15. doi: 10.1007/s13205-019-2013-0
![]() |
[49] |
Wang YB, Zhang WX, Ding CJ, et al. (2019) Endophytic communities of transgenic poplar were determined by the environment and niche rather than by transgenic events. Front Microbiol 10: 588. doi: 10.3389/fmicb.2019.00588
![]() |
[50] |
Mahmood A, Takagi K, Ito K, et al. (2019) Changes in endophytic bacterial communities during different growth stages of cucumber (Cucumis sativus L.). World J Microbiol Biotechnol 35: 104. doi: 10.1007/s11274-019-2676-z
![]() |
[51] |
Hong CE, Kim JU, Lee JW, et al. (2019) Metagenomic analysis of bacterial endophyte community structure and functions in Panax ginseng at different ages. 3 Biotech 9: 300. doi: 10.1007/s13205-019-1838-x
![]() |
[52] |
Santoyo G, Moreno-Hagelsieb G, del Carmen Orozco-Mosqueda M, et al. (2016) Plant growth-promoting bacterial endophytes. Microbiol Res 183: 92-99. doi: 10.1016/j.micres.2015.11.008
![]() |
[53] |
Ding T, Palmer MW, Melcher U (2013) Community terminal restriction fragment length polymorphisms reveal insights into the diversity and dynamics of leaf endophytic bacteria. BMC Microbiol 13: 1. doi: 10.1186/1471-2180-13-1
![]() |
[54] |
Barraza A, Vizuet-de-Rueda JC, Alvarez-Venegas R (2020) Highly diverse root endophyte bacterial community is driven by growth substrate and is plant genotype-independent in common bean (Phaseolus vulgaris L.). Peer J 8: e9423. doi: 10.7717/peerj.9423
![]() |
[55] |
Rashid S, Charles TC, Glick BR (2012) Isolation and characterization of new plant growth-promoting bacterial endophytes. Appl Soil Ecol 61: 217-224. doi: 10.1016/j.apsoil.2011.09.011
![]() |
[56] | Osman KT (2014) Soil degradation, conservation and remediation. Springer. DOI: https://doi.org/10.1007/978-94-007-7590-9. |
[57] |
Tully K, Sullivan C, Weil R, et al. (2015) The state of soil degradation in sub-saharan Africa: baselines, trajectories, and solutions. Sustainability 7: 6523-6552. doi: 10.3390/su7066523
![]() |
[58] |
Lal R (2015) Restoring soil quality to mitigate soil degradation. Sustainability 7: 5875-5895. doi: 10.3390/su7055875
![]() |
[59] |
Bai ZG, Dent DL, Olsson L, et al. (2008) Proxy global assessment of land degradation. Soil Use Manage 24: 223-234. doi: 10.1111/j.1475-2743.2008.00169.x
![]() |
[60] |
Mburu SW, Koskey G, Kimiti JM, et al. (2016) Agrobiodiversity conservation enhances food security in subsistence-based farming systems of Eastern Kenya. Agric Food Secur 5: 19. doi: 10.1186/s40066-016-0068-2
![]() |
[61] |
Nyamwange MM, Njeru EM, Mucheru-Muna M, et al. (2018) Soil management practices affect arbuscular mycorrhizal fungi propagules, root colonization and growth of rainfed maize. AIMS Agric Food 3: 120-134. doi: 10.3934/agrfood.2018.2.120
![]() |
[62] |
Chaer GM, Fernandes MF, Myrold DD, et al. (2009) Shifts in microbial community composition and physiological profiles across a gradient of induced soil degradation. Soil Sci Soc Am J 73: 1327-1334. doi: 10.2136/sssaj2008.0276
![]() |
[63] |
Ahemad M, Kibret M (2014) Mechanisms and applications of plant growth promoting rhizobacteria: Current perspective. J King Saud Univ-Sci 26: 1-20. doi: 10.1016/j.jksus.2013.05.001
![]() |
[64] | Chhabra S, Dowling DN (2017) Endophyte-promoted nutrient acquisition: Phosphorus and iron. In: Doty S. (Ed), Functional importance of the plant microbiome. 21-42. |
[65] | Devi AR, Kotoky R, Pandey P, et al. (2017) Application of Bacillus spp. for sustainable cultivation of potato (Solanum tuberosum L.) and the benefits, In: Islam M, Rahman M, Pandey P. et al. (Eds), Bacilli and Agrobiotechnology. Springer, Cham, 185-211. |
[66] |
Zhalnina K, Louie KB, Hao Z, et al. (2018) Dynamic root exudate chemistry and microbial substrate preferences drive patterns in rhizosphere microbial community assembly. Nat Microbiol 3: 470-480. doi: 10.1038/s41564-018-0129-3
![]() |
[67] | Adesemoye AO, Yuen G, Watts DB (2017) Microbial inoculants for optimized plant nutrient use in integrated pest and input management systems, Probiotics and plant health. Singapore: Springer, 21-40. |
[68] |
Singh D, Geat N, Rajawat MVS, et al. (2018) Prospecting endophytes from different Fe or Zn accumulating wheat genotypes for their influence as inoculants on plant growth, yield, and micronutrient content. Ann Microbiol 68: 815-833. doi: 10.1007/s13213-018-1388-1
![]() |
[69] |
Ku YS, Rehman HM, Lam HM, (2019) Possible roles of rhizospheric and endophytic microbes to provide a safe and affordable means of crop biofortification. Agronomy 9: 764. doi: 10.3390/agronomy9110764
![]() |
[70] | Jamir E, Kangabam RD, Borah K, et al. (2019) Role of soil microbiome and enzyme activities in plant growth nutrition and ecological restoration of soil health. In: Kumar A, Sharma S. (Eds), Microbes and enzymes in soil health and bioremediation. Singapore: Springer, 16: 99-132. |
[71] |
Khare E, Yadav A (2017) The role of microbial enzyme systems in plant growth promotion. Clim Change Environ Sustain 5: 122-145. doi: 10.5958/2320-642X.2017.00013.8
![]() |
[72] |
Nag P, Shriti S, Das S (2020) Microbiological strategies for enhancing biological nitrogen fixation in nonlegumes. J Appl Microbiol 129: 186-198. doi: 10.1111/jam.14557
![]() |
[73] |
Koskey G, Mburu SW, Njeru EM, et al. (2017) Potential of native rhizobia in enhancing nitrogen fixation and yields of climbing beans (Phaseolus vulgaris L.) in contrasting environments of Eastern Kenya. Front Plant Sci 8: 443. doi: 10.3389/fpls.2017.00443
![]() |
[74] |
Kwon SW, Park JY, Kim JS, et al. (2005) Phylogenetic analysis of the genera Bradyrhizobium, Mesorhizobium, Rhizobium and Sinorhizobium on the basis of 16S rRNA gene and internally transcribed spacer region sequences. Int J Syst Evol Microbiol 55: 263-270. doi: 10.1099/ijs.0.63097-0
![]() |
[75] | Tak N, Gehlot HS (2019) Diversity of nitrogen-fixing symbiotic rhizobia with special reference to indian thar desert, In: Satyanarayana T, Das S, Johri B. (Eds), Microbial diversity in ecosystem sustainability and biotechnological applications. Singapore: Springer, 31-55. |
[76] |
Dahal B, NandaKafle G, Perkins L, et al. (2017) Diversity of free-Living nitrogen fixing Streptomyces in soils of the badlands of South Dakota. Microbiol Res 195: 31-39. doi: 10.1016/j.micres.2016.11.004
![]() |
[77] | Njeru EM, Muthini M, Muindi MM, et al. (2020) Exploiting arbuscular mycorrhizal fungi-rhizobia-legume symbiosis to increase smallholder farmers' crop production and resilience under a changing climate. In: Singh B, Safalaoh A, Amuri N, et al. (Eds), Climate impacts on agricultural and natural resource sustainability in Africa. Springer, Cham, 471-488. |
[78] |
Gupta G, Panwar J, Jha PN (2013) Natural occurrence of Pseudomonas aeruginosa, a dominant cultivable diazotrophic endophytic bacterium colonizing Pennisetum glaucum (L.) R. Br. Appl Soil Ecol 64: 252-261. doi: 10.1016/j.apsoil.2012.12.016
![]() |
[79] | Mitran T, Meena RS, Lal R, et al. (2018) Role of soil phosphorus on legume production. In: Meena R, Das A, Yadav G, et al. (Eds), Legumes for soil health and sustainable management. Singapore: Springer, 487-510. |
[80] |
Alori ET, Glick BR, Babalola OO (2017) Microbial phosphorus solubilization and its potential for use in sustainable agriculture. Front Microbiol 8: 971. doi: 10.3389/fmicb.2017.00971
![]() |
[81] |
Penn CJ, Camberato JJ (2019) A critical review on soil chemical processes that control how soil ph affects phosphorus availability to plants. Agriculture 9: 120. doi: 10.3390/agriculture9060120
![]() |
[82] | Walia A, Guleria S, Chauhan A, et al. (2017) Endophytic bacteria: role in phosphate solubilization, In: Maheshwari D, Annapurna K. (Eds), Endophytes: Crop productivity and protection. Springer, Cham, 16: 61-93. |
[83] | Etesami H, Adl SM (2020) Plant growth-promoting rhizobacteria (PGPR) and their action mechanisms in availability of nutrients to plants, In: Kumar M, Kumar V, Prasad R. (Eds), Phyto-Microbiome in Stress Regulation. Singapore: Springer, 147-203. |
[84] |
Kong P, Hong CX (2020) Endophytic Burkholderia sp. SSG as a potential biofertilizer promoting boxwood growth. PeerJ 8: e9547. doi: 10.7717/peerj.9547
![]() |
[85] | dos Santos ML, Berlitz DL, Wiest SLF, et al. (2018) Benefits associated with the interaction of endophytic bacteria and plants. Braz Arch Biol Techn 61: e18160431. |
[86] | Arif MS, Shahzad SM, Yasmeen T, et al. (2017) Improving plant phosphorus (p) acquisition by phosphate-solubilizing bacteria. In: Naeem M, Ansari A, Gill S. (Eds), Essential Plant Nutrients. Springer, Cham, 513-556. |
[87] | Bahadur I, Maurya BR, Kumar A, et al. (2016) Towards the soil sustainability and potassium-solubilizing microorganisms. In: Meena V, Maurya B, Verma J, et al. (Eds), Potassium solubilizing microorganisms for sustainable agriculture. New Delhi: Springer, 255-266. |
[88] |
Minden V, Venterink HO (2019) Plant traits and species interactions along gradients of N, P and K availabilities. Funct Ecol 33: 1611-1626. doi: 10.1111/1365-2435.13387
![]() |
[89] | Kumar A, Bahadur I, Maurya BR (2015) Does a plant growth-promoting rhizobacteria enhance agricultural sustainability. J Pure Appl Microbiol 9: 715-724. |
[90] | Teotia P, Kumar V, Kumar M, et al. (2016) Rhizosphere microbes: Potassium solubilization and crop productivity-present and future aspects. In: Meena V, Maurya B, Verma J, et al. (Eds), Potassium solubilizing microorganisms for sustainable agriculture. New Delhi: Springer, 315-325. |
[91] | Meena VS, Bahadur I, Maurya BR, et al. (2016) Potassium-solubilizing microorganism in evergreen agriculture: An overview. Potassium solubilizing microorganisms for sustainable agriculture. New Delhi: Springer, 1-20. |
[92] | Masood S, Bano A (2016) Mechanism of potassium solubilization in the agricultural soils by the help of soil microorganisms. In: Meena V, Maurya B, Verma J. et al. (Eds), Potassium solubilizing microorganisms for sustainable agriculture. New Delhi: Springer, 137-147. |
[93] | Shrivastava M, Srivastava PC, D'souza SF (2016) KSM soil diversity and mineral solubilization, in relation to crop production and molecular mechanism. In: Meena V, Maurya B, Verma J. et al. (Eds), Potassium solubilizing microorganisms for sustainable agriculture. New Delhi: Springer, 221-234. |
[94] |
Nagpal S, Sharma P, Sirari A, et al. (2020) Coordination of Mesorhizobium sp. and endophytic bacteria as elicitor of biocontrol against fusarium wilt in chickpea. Eur J Plant Pathol 158: 143-161. doi: 10.1007/s10658-020-02062-1
![]() |
[95] | Khan MS, Rizvi A, Saif S, et al. (2017) Phosphate-solubilizing microorganisms in sustainable production of wheat: Current perspective. Probiotics in Agroecosystem. Singapore: Springer, 51-81. |
[96] | Zahedi H (2016) Growth-promoting effect of potassium-solubilizing microorganisms on some crop species, In: Meena V, Maurya B, Verma J. et al. (Eds), Potassium solubilizing microorganisms for sustainable agriculture. New Delhi: Springer, 31-42. |
[97] |
Meena VS, Maurya BR, Verma JP (2014) Does a rhizospheric microorganism enhance K+ availability in agricultural soils? Microbiol Res 169: 337-347. doi: 10.1016/j.micres.2013.09.003
![]() |
[98] |
Granada CE, Passaglia LMP, de Souza EM, et al. (2018) Is phosphate solubilization the forgotten child of plant growth-promoting rhizobacteria? Front Microbiol 9: 2054. doi: 10.3389/fmicb.2018.02054
![]() |
[99] |
Etesami H, Emami S, Alikhani HA (2017) Potassium solubilizing bacteria (KSB): Mechanisms, promotion of plant growth, and future prospects-a review. J Soil Sci Plant Nutr 17: 897-911. doi: 10.4067/S0718-95162017000400005
![]() |
[100] |
Grover M, Ali SZ, Sandhya V, et al. (2011) Role of microorganisms in adaptation of agriculture crops to abiotic stresses. World J Microb Biot 27: 1231-1240. doi: 10.1007/s11274-010-0572-7
![]() |
[101] |
Raza A, Razzaq A, Mehmood SS, et al. (2019) Impact of climate change on crops adaptation and strategies to tackle its outcome: A Review. Plants 8: 34. doi: 10.3390/plants8020034
![]() |
[102] |
Msimbira LA, Smith DL (2020) The roles of plant growth promoting microbes in enhancing plant tolerance to acidity and alkalinity stresses. Front Sustain Food Syst 4: 106. doi: 10.3389/fsufs.2020.00106
![]() |
[103] |
Omomowo OI, Babalola OO (2019) Bacterial and fungal endophytes: Tiny giants with immense beneficial potential for plant growth and sustainable agricultural productivity. Microorganisms 7: 481. doi: 10.3390/microorganisms7110481
![]() |
[104] | Eid AM, Salim SS, Hassan SE, et al. (2019) Role of endophytes in plant health and abiotic stress management. In: Kumar V, Prasad R, Kumar M, et al. (Eds), Microbiome in plant health and disease. Singapore: Springer, 119-144. |
[105] |
Kim N, Jeon HW, Mannaa M, et al. (2019) Induction of resistance against pine wilt disease caused by Bursaphelenchus xylophilus using selected pine endophytic bacteria. Plant Pathol 68: 434-444. doi: 10.1111/ppa.12960
![]() |
[106] |
Gorai PS, Gond SK, Mandal NC (2020) Endophytic microbes and their role to overcome abiotic stress in crop plants. Microbial Serv Restor Ecol 109-122. doi: 10.1016/B978-0-12-819978-7.00008-7
![]() |
[107] |
Vigani G, Rolli E, Marasco R, et al. (2019) Root bacterial endophytes confer drought resistance and enhance expression and activity of a vacuolar H+-pumping pyrophosphatase in pepper plants. Environ Microbiol 21: 3212-3228. doi: 10.1111/1462-2920.14272
![]() |
[108] |
Chandran V, Shaji H, Mathew L (2020) Endophytic microbial influence on plant stress responses. Microb Endophytes 161-193. doi: 10.1016/B978-0-12-819654-0.00007-7
![]() |
[109] |
Dubey A, Malla MA, Kumar A, et al. (2020) Plants endophytes: unveiling hidden agenda for bioprospecting toward sustainable agriculture. Crit Rev Biotechnol 40: 1210-1231. doi: 10.1080/07388551.2020.1808584
![]() |
[110] | Tewari S, Shrivas VL, Hariprasad P, et al. (2019) Harnessing endophytes as biocontrol agents. In: Ansari R, Mahmood I. (Eds), Plant Health Under Biotic Stress. Singapore: Springer, 189-218. |
[111] |
Jiao R, Munir S, He PF, et al. (2020) Biocontrol potential of the endophytic Bacillus amyloliquefaciens YN201732 against tobacco powdery mildew and its growth promotion. Biol Control 143: 104160. doi: 10.1016/j.biocontrol.2019.104160
![]() |
[112] | Berg G, Hallmann J (2007) Control of plant pathogenic fungi with bacterial endophytes. Microbial Root Endophytes. In: Schulz BJE, Boyle CJC, Sieber TN. (Eds), Heidelberg: Springer, 9: 53-69. |
[113] |
Beneduzi A, Ambrosini A, Passaglia LMP (2012) Plant growth-promoting rhizobacteria (PGPR): Their potential as antagonists and biocontrol agents. Genet Mol Biol 35: 1044-1051. doi: 10.1590/S1415-47572012000600020
![]() |
[114] |
Hashem A, Tabassum B, Fathi Abd_Allah E (2019) Bacillus subtilis: A plant-growth promoting rhizobacterium that also impacts biotic stress. Saudi J Biol Sci 26: 1291-1297. doi: 10.1016/j.sjbs.2019.05.004
![]() |
[115] | Wang T, Li F, Lu Q, et al. (2020) Studies on diversity, novelty, antimicrobial activity, and new antibiotics of cultivable endophytic actinobacteria isolated from psammophytes collected in Taklamakan Desert. J Pharm Anal. In Press. |
[116] |
Cassán F, Vanderleyden J, Spaepen S (2014) Physiological and agronomical aspects of phytohormone production by model plant-growth-promoting rhizobacteria (pgpr) belonging to the genus Azospirillum. J Plant Growth Regul 33: 440-459. doi: 10.1007/s00344-013-9362-4
![]() |
[117] |
Vardharajula S, SkZ A, Krishna Prasad Vurukonda SS, et al. (2017) Plant growth promoting endophytes and their interaction with plants to alleviate abiotic stress. Curr Biotechnol 6. doi: 10.2174/2211550106666161226154619
![]() |
[118] |
Chaparro JM, Badri DV, Vivanco JM (2014) Rhizosphere microbiome assemblage is affected by plant development. ISME J 8: 790-803. doi: 10.1038/ismej.2013.196
![]() |
[119] | Rajput RS, Ram RM, Vaishnav A, et al. (2019) Microbe-based novel biostimulants for sustainable crop production. In: Satyanarayana T, Das S, Johri B. (Eds), Microbial diversity in ecosystem sustainability and biotechnological applications. Singapore: Springer, 2: 109-144. |
[120] | Chaudhary T, Shukla P (2020) Commercial bioinoculant development: Techniques and challenges. In: Shukla P. (Eds), Microbial enzymes and biotechniques. Singapore: Springer, 57-70. |
[121] |
Timmusk S, Behers L, Muthoni J, et al. (2017) Perspectives and challenges of microbial application for crop improvement. Front Plant Sci 8: 49. doi: 10.3389/fpls.2017.00049
![]() |
[122] | Lesueur D, Deaker R, Herrmann L, et al. (2016) The production and potential of biofertilizers to improve crop. Bioformulations: For sustainable agriculture. In: Arora N, Mehnaz S, Balestrini R. (Eds), New Delhi: Springer, 71-92. |
[123] | Chatterjee R, Roy A, Thirumdasu RK (2017) Microbial inoculants in organic vegetable production: Current perspective. Microbial strategies for vegetable production, Springer International Publishing, 1-21. |
[124] |
Fan B, Wang C, Song XF, et al. (2018) Bacillus velezensis FZB42 in 2018: The gram-positive model strain for plant growth promotion and biocontrol. Front Microbiol 9: 2491. doi: 10.3389/fmicb.2018.02491
![]() |
[125] |
Moawad H, Abd El-Rahim WM, Abd El-Aleem D, et al. (2005) Persistence of two Rhizobium etli inoculant strains in clay and silty loam soils. J Basic Microbiol 45: 438-446. doi: 10.1002/jobm.200510590
![]() |
[126] | Krober M, Wibberg D, Grosch R, et al. (2014) Effect of the strain Bacillus amyloliquefaciens FZB42 on the microbial community in the rhizosphere of lettuce under field conditions analyzed by whole metagenome sequencing. Front Microbiol 5: 252. |
[127] |
Bashan Y, de-Bashan LE, Prabhu SR, et al. (2014) Advances in plant growth-promoting bacterial inoculant technology: Formulations and practical perspectives (1998-2013). Plant Soil 378: 1-33. doi: 10.1007/s11104-013-1956-x
![]() |
[128] |
Stella M, Theeba M, Illani ZI, et al. (2019) Organic fertilizer amended with immobilized bacterial cells for extended shelf-life. Biocatal Agric Biotechnol 20: 101248. doi: 10.1016/j.bcab.2019.101248
![]() |
[129] |
Parnell JJ, Berka R, Young HA, et al. (2016) From the lab to the farm: An industrial perspective of plant beneficial microorganisms. Front Plant Sci 7: 1110. doi: 10.3389/fpls.2016.01110
![]() |
[130] |
Vanlauwe B, Hungria M, Kanampiu F, et al. (2019) The role of legumes in the sustainable intensification of African smallholder agriculture: Lessons learnt and challenges for the future. Agric Ecosyst Environ 284: 106583. doi: 10.1016/j.agee.2019.106583
![]() |
[131] |
Lally RD, Galbally P, Moreira AS, et al. (2017) Application of endophytic pseudomonas fluorescens and a bacterial consortium to Brassica napus can increase plant height and biomass under greenhouse and field conditions. Front Plant Sci 8: 2193. doi: 10.3389/fpls.2017.02193
![]() |
[132] |
ALKahtani MDF, Fouda A, Attia KA, et al. (2020) Isolation and characterization of plant growth promoting endophytic bacteria from desert plants and their application as bioinoculants for sustainable agriculture. Agronomy 10: 1325. doi: 10.3390/agronomy10091325
![]() |
[133] |
Emami S, Alikhani HA, Pourbabaei AA, et al. (2019) Effect of rhizospheric and endophytic bacteria with multiple plant growth promoting traits on wheat growth. Environ Sci Pollut Res 26: 19804-19813. doi: 10.1007/s11356-019-05284-x
![]() |
[134] |
Abiala MA, Odebode AC (2015) Rhizospheric Enterobacter enhanced maize seedling health and growth. Biocontrol Sci Techn 25: 359-372. doi: 10.1080/09583157.2014.981248
![]() |
[135] |
Tagele SB, Kim SW, Lee HG, et al. (2019) Potential of novel sequence Type of Burkholderia cenocepacia for biological control of root rot of maize (Zea mays L.) Caused by Fusarium temperatum. Int J Mol Sci 20: 1005. doi: 10.3390/ijms20051005
![]() |
[136] |
Rfaki A, Zennouhi O, Aliyat FZ, et al. (2020) Isolation, selection and characterization of root-associated rock phosphate solubilizing bacteria in moroccan wheat (Triticum aestivum L.). Geomicrobiol J 37: 230-241. doi: 10.1080/01490451.2019.1694106
![]() |
[137] |
Macedo-Raygoza GM, Valdez-Salas B, Prado FM, et al. (2019) Enterobacter cloacae, an endophyte that establishes a nutrient-transfer symbiosis with banana plants and protects against the black sigatoka pathogen. Front Microbiol 10: 804. doi: 10.3389/fmicb.2019.00804
![]() |
[138] |
Kumar K, Pal G, Verma A, et al. (2020) Seed inhabiting bacterial endophytes of finger millet (Eleusine coracana L.) promote seedling growth and development, and protect from fungal disease. S Afr J Bot 134: 91-98. doi: 10.1016/j.sajb.2020.03.032
![]() |
[139] |
Panigrahi S, Mohanty S, Rath CC (2019) Characterization of endophytic bacteria Enterobacter cloacae MG00145 isolated from Ocimum sanctum with Indole Acetic Acid (IAA) production and plant growth promoting capabilities against selected crops. S Afr J Bot 134: 17-26. doi: 10.1016/j.sajb.2019.09.017
![]() |
[140] |
Yaish MW, Antony I, Glick BR (2015) Isolation and characterization of endophytic plant growth-promoting bacteria from date palm tree (Phoenix dactylifera L.) and their potential role in salinity tolerance. Antonie Van Leeuwenhoek 107: 1519-1532. doi: 10.1007/s10482-015-0445-z
![]() |
[141] |
Neelipally RTKR, Anoruo AO, Nelson S (2020) Effect of Co-Inoculation of Bradyrhizobium and Trichoderma on growth, development, and yield of Arachis hypogaea L. (Peanut). Agronomy 10: 1415. doi: 10.3390/agronomy10091415
![]() |
[142] |
Shifa H, Gopalakrishnan C, Velazhahan R (2014) Efficacy of Bacillus subtilis G-1 in suppression of stem rot caused by Sclerotium rolfsii and growth promotion of groundnut. Int J Agric Environ Biotechnol 8: 111. doi: 10.5958/2230-732X.2015.00015.7
![]() |
[143] |
Adhikari A, Dutta S, Chattopadhaya A, et al. (2013) Potential effects of plant growth promoting rhizobacteria (Pseudomonas fluorescens) on cowpea seedling health and damping off disease control. Afr J Biotechnol 12: 1853-1861. doi: 10.5897/AJB12.2846
![]() |
[144] |
Udaya Shankar AC, Nayaka SC, Niranjan-Raj S, et al. (2009) Rhizobacteria-mediated resistance against the blackeye cowpea mosaic strain of bean common mosaic virus in cowpea (Vigna unguiculata). Pest Manag Sci 65: 1059-1064. doi: 10.1002/ps.1791
![]() |
[145] |
Bedine Boat MA, Sameza ML, Iacomi B, et al. (2020) Screening, identification and evaluation of Trichoderma spp. for biocontrol potential of common bean damping-off pathogens. Biocontrol Sci Technol 30: 228-242. doi: 10.1080/09583157.2019.1700909
![]() |
[146] |
Sendi Y, Pfeiffer T, Koch E, et al. (2020) Potential of common bean (Phaseolus vulgaris L.) root microbiome in the biocontrol of root rot disease and traits of performance. J Plant Dis Prot 127: 453-462. doi: 10.1007/s41348-020-00338-6
![]() |
[147] |
Sabaté DC, Brandan CP, Petroselli G, et al. (2017) Decrease in the incidence of charcoal root rot in common bean (Phaseolus vulgaris L.) by Bacillus amyloliquefaciens B14, a strain with PGPR properties. Biol Control 113: 1-8. doi: 10.1016/j.biocontrol.2017.06.008
![]() |
[148] |
Mburu SW, Koskey G, Njeru EM, et al. (2020) Differential response of promiscuous soybean to local diversity of indigenous and commercial Bradyrhizobium inoculation under contrasting agroclimatic sones. Int J Plant Prod 14: 578-582. doi: 10.1007/s42106-020-00117-1
![]() |
[149] |
Khan MA, Asaf S, Khan AL, et al. (2020) Thermotolerance effect of plant growth-promoting Bacillus cereus SA1 on soybean during heat stress. BMC Microbiol 20: 175. doi: 10.1186/s12866-020-01822-7
![]() |
[150] |
El-Esawi MA, Alaraidh IA, Alsahli AA, et al. (2018) Bacillus firmus (SW5) augments salt tolerance in soybean (Glycine max L.) by modulating root system architecture, antioxidant defense systems and stress-responsive genes expression. Plant Physiol Bioch 132: 375-384. doi: 10.1016/j.plaphy.2018.09.026
![]() |
[151] |
Yasmin H, Naeem S, Bakhtawar M, et al. (2020) Halotolerant rhizobacteria Pseudomonas pseudoalcaligenes and Bacillus subtilis mediate systemic tolerance in hydroponically grown soybean (Glycine max L.) against salinity stress. PLoS One 15: e0231348. doi: 10.1371/journal.pone.0231348
![]() |
[152] |
Chinheya CC, Yobo KS, Laing MD (2017) Biological control of the rootknot nematode, Meloidogyne javanica (Chitwood) using Bacillus isolates, on soybean. Biol Control 109: 37-41. doi: 10.1016/j.biocontrol.2017.03.009
![]() |
[153] |
Freitas MA, Medeiros FHV, Melo IS, et al. (2019) Stem inoculation with bacterial strains Bacillus amyloliquefaciens (GB03) and Microbacterium imperiale (MAⅡF2a) mitigates Fusarium root rot in cassava. Phytoparasitica 47: 135-142. doi: 10.1007/s12600-018-0706-2
![]() |
[154] |
Chaintreuil C, Giraud E, Prin Y, et al. (2000) Photosynthetic bradyrhizobia are natural endophytes of the African wild rice Oryza breviligulata. Appl Environ Microbiol 66: 5437-5447. doi: 10.1128/AEM.66.12.5437-5447.2000
![]() |
[155] |
Rania ABA, Jabnoun-Khiareddine H, Nefzi A, et al. (2016) Endophytic bacteria from Datura metel for plant growth promotion and bioprotection against Fusarium wilt in tomato. Biocontrol Sci Technol 26: 1139-1165. doi: 10.1080/09583157.2016.1188264
![]() |
[156] |
Eke P, Kumar A, Sahu KP, et al. (2019) Endophytic bacteria of desert cactus (Euphorbia trigonas Mill) confer drought tolerance and induce growth promotion in tomato (Solanum lycopersicum L.). Microbiol Res 228: 126302. doi: 10.1016/j.micres.2019.126302
![]() |
[157] |
Khan MA, Asaf S, Khan AL, et al. (2020) Extending thermotolerance to tomato seedlings by inoculation with SA1 isolate of Bacillus cereus and comparison with exogenous humic acid application. PLoS One 15: e0232228. doi: 10.1371/journal.pone.0232228
![]() |
[158] |
Kumar V, Jain L, Jain SK, et al. (2020) Bacterial endophytes of rice (Oryza sativa L.) and their potential for plant growth promotion and antagonistic activities. S Afr J Bot 134: 50-63. doi: 10.1016/j.sajb.2020.02.017
![]() |
[159] |
Vargas L, Brígida ABS, Mota Filho JP, et al. (2014) Drought tolerance conferred to sugarcane by association with Gluconacetobacter diazotrophicus: A transcriptomic view of hormone pathways. PLoS One 9: e114744. doi: 10.1371/journal.pone.0114744
![]() |
[160] |
Wang CJ, Wang YZ, Chu ZH, et al. (2020) Endophytic Bacillus amyloliquefaciens YTB1407 elicits resistance against two fungal pathogens in sweet potato (Ipomoea batatas (L.) Lam.). J Plant Physiol 253: 153260. doi: 10.1016/j.jplph.2020.153260
![]() |
[161] |
Ambrosini A, Beneduzi A, Stefanski T, et al. (2012) Screening of plant growth promoting Rhizobacteria isolated from sunflower (Helianthus annuus L.). Plant Soil 356: 245-264. doi: 10.1007/s11104-011-1079-1
![]() |
[162] |
Yoon V, Tian G, Vessey JK, et al. (2016) Colonization efficiency of different sorghum genotypes by Gluconacetobacter diazotrophicus. Plant Soil 398: 243-256. doi: 10.1007/s11104-015-2653-8
![]() |
[163] |
Schlemper TR, Dimitrov MR, Gutierrez FAOS, et al. (2018) Effect of Burkholderia tropica and Herbaspirillum frisingense strains on sorghum growth is plant genotype dependent. PeerJ 2018: e5346. doi: 10.7717/peerj.5346
![]() |
[164] |
Majeed A, Abbasi MK, Hameed S, et al. (2015) Isolation and characterization of plant growth-promoting rhizobacteria from wheat rhizosphere and their effect on plant growth promotion. Front Microbiol 6: 198. doi: 10.3389/fmicb.2015.00198
![]() |
[165] |
Dahal RH, Chaudhary DK, Kim DU, et al. (2021) Chryseobacterium antibioticum sp. nov. with antimicrobial activity against Gram-negative bacteria, isolated from Arctic soil. J Antibiot (Tokyo) 74: 115-123. doi: 10.1038/s41429-020-00367-1
![]() |
[166] |
Chen YH, Yang XZ, Li Z, et al. (2020) Efficiency of potassium-solubilizing Paenibacillus mucilaginosus for the growth of apple seedling. J Integr Agric 19: 2458-2469. doi: 10.1016/S2095-3119(20)63303-2
![]() |
[167] |
Breitkreuz C, Buscot F, Tarkka M, et al. (2020) Shifts between and among populations of wheat rhizosphere Pseudomonas, Streptomyces and Phyllobacterium suggest consistent phosphate mobilization at different wheat growth stages under abiotic stress. Front Microbiol 10: 3109. doi: 10.3389/fmicb.2019.03109
![]() |
[168] |
Yasmin S, Hafeez FY, Mirza MS, et al. (2017) Biocontrol of Bacterial Leaf Blight of rice and profiling of secondary metabolites produced by rhizospheric Pseudomonas aeruginosa BRp3. Front Microbiol 8: 1895. doi: 10.3389/fmicb.2017.01895
![]() |
[169] |
Xu S, Xie XW, Zhao YR, et al. (2020) Whole-genome analysis of Bacillus velezensis ZF2, a biocontrol agent that protects cucumis sativus against corynespora leaf spot diseases. 3 Biotech 10: 186. doi: 10.1007/s13205-020-2165-y
![]() |
[170] |
Tewari S, Arora NK (2016) Fluorescent Pseudomonas sp. PF17 as an efficient plant growth regulator and biocontrol agent for sunflower crop under saline conditions. Symbiosis 68: 99-108. doi: 10.1007/s13199-016-0389-8
![]() |
[171] | Raffi MM, Charyulu PBBN (2021) Azospirillum-biofertilizer for sustainable cereal crop production: Current status. Recent developments in applied microbiology and biochemistry. Elsevier, 193-209. |
[172] |
Nayak AK, Panda SS, Basu A, et al. (2018) Enhancement of toxic Cr (Ⅵ), Fe, and other heavy metals phytoremediation by the synergistic combination of native Bacillus cereus strain and Vetiveria zizanioides L. Int J Phytoremediation 20: 682-691. doi: 10.1080/15226514.2017.1413332
![]() |
[173] |
Aloo BN, Makumba BA, Mbega ER (2019) The potential of Bacilli rhizobacteria for sustainable crop production and environmental sustainability. Microbiol Res 219: 26-39. doi: 10.1016/j.micres.2018.10.011
![]() |
[174] |
Wu SH, Zhuang GQ, Bai ZH, et al. (2018) Mitigation of nitrous oxide emissions from acidic soils by Bacillus amyloliquefaciens, a plant growth-promoting bacterium. Glob Chang Biol 24: 2352-2365. doi: 10.1111/gcb.14025
![]() |
[175] |
dos Santos CLR, Alves GC, de Matos Macedo AV, et al. (2017) Contribution of a mixed inoculant containing strains of Burkholderia spp. and Herbaspirillum ssp. to the growth of three sorghum genotypes under increased nitrogen fertilization levels. Appl Soil Ecol 113: 96-106. doi: 10.1016/j.apsoil.2017.02.008
![]() |
[176] |
Lourenço KS, Cassman NA, Pijl AS, et al. (2018) Nitrosospira sp. govern nitrous oxide emissions in a tropical soil amended with residues of bioenergy crop. Front Microbiol 9: 674. doi: 10.3389/fmicb.2018.00674
![]() |
[177] |
Patel P, Trivedi G, Saraf M (2018) Iron biofortification in mungbean using siderophore producing plant growth promoting bacteria. Environ Sustain 1: 357-365. doi: 10.1007/s42398-018-00031-3
![]() |
[178] |
Arya N, Rana A, Rajwar A, et al. (2018) Biocontrol efficacy of siderophore producing indigenous Pseudomonas strains against fusarium wilt in tomato. Natl Acad Sci Lett 41: 133-136. doi: 10.1007/s40009-018-0630-5
![]() |
[179] | Ong KS, Aw YK, Lee LH, et al. (2016) Burkholderia paludis sp. nov., an antibiotic-siderophore producing novel Burkholderia cepacia complex species, isolated from Malaysian Tropical Peat Swamp Soil. Front Microbiol 7: 2046. |
[180] |
Jung BK, Hong SJ, Park GS, et al. (2018) Isolation of Burkholderia cepacia JBK9 with plant growth-promoting activity while producing pyrrolnitrin antagonistic to plant fungal diseases. Appl Biol Chem 61: 173-180. doi: 10.1007/s13765-018-0345-9
![]() |
[181] |
Hoffmann MC, Müller A, Fehringer M, et al. (2014) Coordinated Expression of fdxD and molybdenum nitrogenase genes promotes nitrogen fixation by Rhodobacter capsulatus in the presence of oxygen. J Bacteriol 196: 633-640. doi: 10.1128/JB.01235-13
![]() |
[182] | Mathur A, Koul A, Hattewar J (2019) Plant growth-promoting rhizobacteria (pgprs): significant revolutionary tools for achieving long-term sustainability and combating the biotic stress caused by the attack of pathogens affecting crops in agriculture, In: Sayyed R. (Ed), Plant growth promoting rhizobacteria for sustainable stress management. Singapore: Springer, 379-388. |
[183] |
de Lima DRM, dos Santos IB, Oliveira JTC, et al. (2020) Genetic diversity of N-fixing and plant growth-promoting bacterial community in different sugarcane genotypes, association habitat and phenological phase of the crop. Arch Microbiol 203: 1089-1105. doi: 10.1007/s00203-020-02103-7
![]() |
[184] |
Soenens A, Imperial J (2020) Biocontrol capabilities of the genus Serratia. Phytochem Rev 19: 577-587. doi: 10.1007/s11101-019-09657-5
![]() |
[185] | Sasirekha B, Srividya S (2016) Siderophore production by Pseudomonas aeruginosa FP6, a biocontrol strain for Rhizoctonia solani and Colletotrichum gloeosporioides causing diseases in chilli. Agric Nat Resour 50: 250-256. |
1. | Xiaohe Song, Yankai Li, Yong Hu, Weidong Guo, Zirui Wu, Yao Zhang, Zhe Cao, Endophytes from blueberry roots and their antifungal activity and plant growth enhancement effects, 2021, 20, 24522198, 100454, 10.1016/j.rhisph.2021.100454 | |
2. | Simon W. Mburu, Gilbert Koskey, Ezekiel M. Njeru, Omwoyo Ombori, John Maingi, Jacinta M. Kimiti, Genetic and phenotypic diversity of microsymbionts nodulating promiscuous soybeans from different agro-climatic conditions, 2022, 20, 2090-5920, 10.1186/s43141-022-00386-5 | |
3. | Lavinia Diana Nicoleta Barbu, Oana-Alina Boiu-Sicuia, "PLANT-BENEFICIAL MICROBIAL INOCULANTS AND THEIR FORMULATION – A REVIEW", 2021, 14, 2248129X, 32, 10.54574/RJPP.14.05 | |
4. | Vagish Dwibedi, Santosh Kumar Rath, Mahavir Joshi, Rajinder Kaur, Gurleen Kaur, Davinder Singh, Gursharan Kaur, SukhminderJit Kaur, Microbial endophytes: application towards sustainable agriculture and food security, 2022, 106, 0175-7598, 5359, 10.1007/s00253-022-12078-8 | |
5. | Jishma Panichikkal, Ashitha Jose, Sreejith Sreekumaran, Anju Kanjirakandi Ashokan, Cimmiya Susan Baby, Radhakrishnan Edayileveetil Krishnankutty, Biofilm and Biocontrol Modulation of Paenibacillus sp. CCB36 by Supplementation with Zinc Oxide Nanoparticles and Chitosan Nanoparticles, 2022, 194, 0273-2289, 1606, 10.1007/s12010-021-03710-w | |
6. | Shifa Shaffique, Muhammad Aaqil Khan, Shabir Hussain Wani, Anjali Pande, Muhammad Imran, Sang-Mo Kang, Waqas Rahim, Sumera Afzal Khan, Dibya Bhatta, Eun-Hae Kwon, In-Jung Lee, A Review on the Role of Endophytes and Plant Growth Promoting Rhizobacteria in Mitigating Heat Stress in Plants, 2022, 10, 2076-2607, 1286, 10.3390/microorganisms10071286 | |
7. | Adekunle Raimi, Rasheed Adeleke, 16S rRNA gene‐based identification and plant growth‐promoting potential of cultivable endophytic bacteria, 2023, 115, 0002-1962, 1447, 10.1002/agj2.21241 | |
8. | Goshu Misganaw, 2024, Chapter 140-1, 978-3-030-98067-2, 1, 10.1007/978-3-030-98067-2_140-1 | |
9. | Tiwit Widowati, Liseu Nurjanah, Rumella Simarmata, Sylvia J. R. Lekatompessy, 2023, 2972, 0094-243X, 030003, 10.1063/5.0183210 | |
10. | Maqsood Ahmed Khaskheli, Mir Muhammad Nizamani, Entaj Tarafder, Diptosh Das, Ghulam Muhae-Ud-Din, Raheel Ahmed Khaskheli, Yong Wang, Manipulation of Root-Associated Bacterial Endophytes for Sustainable Crop Production System: A Review, 2025, 24522198, 101044, 10.1016/j.rhisph.2025.101044 | |
11. | María Paz Villanueva-Llanes, María Carbú, Jesús Manuel Cantoral, Gustavo Cordero-Bueso, Exploring the microbiota of grapevines: tools and perspectives for sustainable agriculture, 2025, 26661543, 101795, 10.1016/j.jafr.2025.101795 |
Crop | Endophytic bacteria | Bioactive activity | References |
Maize (Zea mays) | Enterobacter spp. | Disease suppression (Against Fusarium verticillioides) Seedling growth and health | [134] |
Burkholderia spp. | Enhance growth and P-utilization | [135] | |
Wheat | Enterobactereacea (Pantoea genus) | IAA and siderophore producer, and also solubilised phosphate biocontrol abilities against Fusarium graminearum | [136] |
Banana (Musa spp.) | Enterobacter cloacae | Promote growth and health | [137] |
Finger millet (Eleusine coracona (L). Gaertner) | Pseudomonas spp. | Blast disease management and growth promotion | [138] |
Oryza sativa (rice), Arachis hypogaea (groundnut), Vigna mungo (black gram) | Enterobacter cloacae | Enhance seed germination index, shoot and root biomass of seedling, seed vigour index and salinity tolerance | [139,140] |
Peanut (Arachis hypogaea L.) | Bradyrhizobium and Trichoderma | Growth improvement | [141] |
Groundnut (Arachis hypogaea L.) | Bacillus subtilis | Suppression of stem rot caused by Sclerotium rolfsii and growth promotion | [142] |
Cowpea (Vigna unguiculata) | Pseudomonas fluorescens | Seed health and yield | [143] |
Damping off control caused by Sclerotium rolfsii | |||
Bacillus pumilus and Bacillus subtilis | Resistance against the black eye cowpea mosaic strain | [144] | |
Common Bean (Phaseolus vulgaris L.) | Trichoderma atroviridae | Biocontrol against common bean root rot (Fusarium solani) and growth enhancement. | [145] |
Rhizobium | Nitrogen (N) fixation | ||
Bacillus spp. | Biocontrol activity of Fusarium sp., Macrophomina sp., and Alternaria sp | [146] | |
Bacillus amyloliquefaciens | Biocontrol against charcoal root rot | [147] | |
Soybean (Glycine max) | Bradyrhizobium | Nitrogen fixation | [148] |
Bacillus cereus | Mitigation of heat stress damage | [149] | |
Bacillus firmus (SW5) | Enhancing salt tolerance | [150] | |
Pseudomonas koreensis | Salt sress tolerance in soybean | ||
Pseudomonas pseudoalcaligenes | Alleviates salt stress in soybean plants | [151] | |
Bacillus spp. | Biological control of the rootknot nematode, Meloidogyne javanica (Chitwood) | [152] | |
Cassava | Bacillus amyloliquefaciens and Microbacterium imperiale | Mitigates Fusarium root rot disease | [153] |
African cultivated rice (Oryza glaberrima) | Photosynthetic Bradyrhizobium | Increase in the shoot growth and grain yield | [154] |
Tomato | Stenotrophomonas sp. str. S33 (KR818084) and Pseudomonas sp | Suppress tomato Fusarium wilt disease caused by Fusarium oxysporum f. sp. Lycopersici (FOL) | [155] |
Bacillus amyloliquefaciens | Drought tolerance | [156] | |
Bacillus cereus | Thermotolerance | [157] | |
Rice (Oryza sativa) | Bacillus subtilis | Disease suppression against fungal pathogens Rhizoctonia solani, Fusarium verticelloides and Sclerotium rolfsii.antibacterial activities against Xanthomonas oryzae | [158] |
Sugracane (Saccharum officinarum) | Gluconacetobacter diazotrophicus | Drought tolerance | [159] |
Date palm tree (Phoenix dactylifera L.) | Paenibacillus xylanexedens) and (Enterobacter cloacae) | Facilitate nutrient uptake in roots enhance canola root elongation | [140] |
Sweet potato (Ipomoea batatas (L.) Lam.) | Bacillus amyloliquefaciens | Resistance against fungal pathogens | [160] |
Sunflower (Helianthus annuus L.) | Burkholderia | Stimulate plant growth | [161] |
Sorghum (Sorghum bicolor) | Gluconacetobacter diazotrophicus | Nitrogen fixation | [162] |
Burkholderia tropica | Growth promotion | [163] |
Rhizobacteria | Growth promotion traits | Associated rhizosphere | References |
Stenotrophomonas spp | Enhance nutrients uptake and growth | Wheat (Triticum aestivum L.) | [164] |
Chryseobacterium antibioticum | Antimicrobial activity against gram-negative bacteria.Siderophore production | Arctic soil | [165] |
Gluconacetobacter diazotrophicus | Drought tolerance Nitrogen fixation | Sugarcane (Saccharum officinarum) | [159] |
Paenibacillus mucilaginosus | IAA production Potassium solubilization | Apple (Malus domestica) | [166] |
Phyllobacterium ifriqiyense and Phyllobacterium sophorae | Siderophore production Phosphate solubilization | Wheat (Triticum aestivum L.) | [167] |
Pseudomonas aeruginosa | Biocontrol agents against bacterial blight disease Stress management through glucanase and chitinase production | Rice (Oryza sativa) | [168] |
Bacillus velezensis | Biocontrol agent against corynespora leaf spot diseases | Cucumber (Cucumis sativus) | [169] |
Pseudomonas sp | Salinity tolerance | Sunflower (Helianthus annuus) | [170] |
Azospirillum | Biofertilizer | Barley (Hordeum vulgare), wheat (Triticum aestivum L.), oats | [171] |
Bacillus cereus | Phytoremediation of heavy metals | Vetiveria zizanioides L | [172] |
Bacillus spp. | Auxin synthesis Production of antibiotics, siderophores and enzymes | Potato (Solanum tuberosum), cucumber (Cucumis sativus), peanuts (Arachis hypogaea) | [173] |
Bacillus amyloliquefaciens | Mitigation of nitrous oxide emissions | Acidic soils | [174] |
Burkholderia kururiensis | Nutrients uptake Nitrogen fixation | Sorghum (Sorghum bicolor) | [175] |
Nitrosospira spp. | Management and control of nitrous oxide in soils with excess use of inorganic fertilizers | Tropical soils | [176] |
Pseudomonas putida | Iron translocation through siderophore production | Mung bean (Vigna radiata) | [177] |
Pseudomonas fluorescens | Biocontrol agent against fusarium wilt disease | Tomato (Solanum lycopersicum) | [178] |
Burkholderia paludis | Siderophore and antibiotic production | Forest rhizosphere | [179] |
Bacillus cepacia | Biocontrol agent against fungal pathogens | Pepper plant (Piper nigrum) | [180] |
Rhodobacteria | Nitrogen fixation | Wheat (Triticum aestivum L.) | [181] |
Azarcus | Nitrogen fixation | Rice (Oryza sativa) | [182] |
Azorhizobium | Nitrogen fixation | Sugarcane (Saccharum officinarum) | [183] |
SerratiaMarcescens | Pathogen biocontrol Antifungal | Wheat (Triticum aestivum L.) | [184] |
Pseudomonas aeruginosa | Siderophore production Biocontrol agent against Rhizoctonia solani and Colletotrichum gloeosporioides | Chilli (Capsicum frutescens) | [185] |
Crop | Endophytic bacteria | Bioactive activity | References |
Maize (Zea mays) | Enterobacter spp. | Disease suppression (Against Fusarium verticillioides) Seedling growth and health | [134] |
Burkholderia spp. | Enhance growth and P-utilization | [135] | |
Wheat | Enterobactereacea (Pantoea genus) | IAA and siderophore producer, and also solubilised phosphate biocontrol abilities against Fusarium graminearum | [136] |
Banana (Musa spp.) | Enterobacter cloacae | Promote growth and health | [137] |
Finger millet (Eleusine coracona (L). Gaertner) | Pseudomonas spp. | Blast disease management and growth promotion | [138] |
Oryza sativa (rice), Arachis hypogaea (groundnut), Vigna mungo (black gram) | Enterobacter cloacae | Enhance seed germination index, shoot and root biomass of seedling, seed vigour index and salinity tolerance | [139,140] |
Peanut (Arachis hypogaea L.) | Bradyrhizobium and Trichoderma | Growth improvement | [141] |
Groundnut (Arachis hypogaea L.) | Bacillus subtilis | Suppression of stem rot caused by Sclerotium rolfsii and growth promotion | [142] |
Cowpea (Vigna unguiculata) | Pseudomonas fluorescens | Seed health and yield | [143] |
Damping off control caused by Sclerotium rolfsii | |||
Bacillus pumilus and Bacillus subtilis | Resistance against the black eye cowpea mosaic strain | [144] | |
Common Bean (Phaseolus vulgaris L.) | Trichoderma atroviridae | Biocontrol against common bean root rot (Fusarium solani) and growth enhancement. | [145] |
Rhizobium | Nitrogen (N) fixation | ||
Bacillus spp. | Biocontrol activity of Fusarium sp., Macrophomina sp., and Alternaria sp | [146] | |
Bacillus amyloliquefaciens | Biocontrol against charcoal root rot | [147] | |
Soybean (Glycine max) | Bradyrhizobium | Nitrogen fixation | [148] |
Bacillus cereus | Mitigation of heat stress damage | [149] | |
Bacillus firmus (SW5) | Enhancing salt tolerance | [150] | |
Pseudomonas koreensis | Salt sress tolerance in soybean | ||
Pseudomonas pseudoalcaligenes | Alleviates salt stress in soybean plants | [151] | |
Bacillus spp. | Biological control of the rootknot nematode, Meloidogyne javanica (Chitwood) | [152] | |
Cassava | Bacillus amyloliquefaciens and Microbacterium imperiale | Mitigates Fusarium root rot disease | [153] |
African cultivated rice (Oryza glaberrima) | Photosynthetic Bradyrhizobium | Increase in the shoot growth and grain yield | [154] |
Tomato | Stenotrophomonas sp. str. S33 (KR818084) and Pseudomonas sp | Suppress tomato Fusarium wilt disease caused by Fusarium oxysporum f. sp. Lycopersici (FOL) | [155] |
Bacillus amyloliquefaciens | Drought tolerance | [156] | |
Bacillus cereus | Thermotolerance | [157] | |
Rice (Oryza sativa) | Bacillus subtilis | Disease suppression against fungal pathogens Rhizoctonia solani, Fusarium verticelloides and Sclerotium rolfsii.antibacterial activities against Xanthomonas oryzae | [158] |
Sugracane (Saccharum officinarum) | Gluconacetobacter diazotrophicus | Drought tolerance | [159] |
Date palm tree (Phoenix dactylifera L.) | Paenibacillus xylanexedens) and (Enterobacter cloacae) | Facilitate nutrient uptake in roots enhance canola root elongation | [140] |
Sweet potato (Ipomoea batatas (L.) Lam.) | Bacillus amyloliquefaciens | Resistance against fungal pathogens | [160] |
Sunflower (Helianthus annuus L.) | Burkholderia | Stimulate plant growth | [161] |
Sorghum (Sorghum bicolor) | Gluconacetobacter diazotrophicus | Nitrogen fixation | [162] |
Burkholderia tropica | Growth promotion | [163] |
Rhizobacteria | Growth promotion traits | Associated rhizosphere | References |
Stenotrophomonas spp | Enhance nutrients uptake and growth | Wheat (Triticum aestivum L.) | [164] |
Chryseobacterium antibioticum | Antimicrobial activity against gram-negative bacteria.Siderophore production | Arctic soil | [165] |
Gluconacetobacter diazotrophicus | Drought tolerance Nitrogen fixation | Sugarcane (Saccharum officinarum) | [159] |
Paenibacillus mucilaginosus | IAA production Potassium solubilization | Apple (Malus domestica) | [166] |
Phyllobacterium ifriqiyense and Phyllobacterium sophorae | Siderophore production Phosphate solubilization | Wheat (Triticum aestivum L.) | [167] |
Pseudomonas aeruginosa | Biocontrol agents against bacterial blight disease Stress management through glucanase and chitinase production | Rice (Oryza sativa) | [168] |
Bacillus velezensis | Biocontrol agent against corynespora leaf spot diseases | Cucumber (Cucumis sativus) | [169] |
Pseudomonas sp | Salinity tolerance | Sunflower (Helianthus annuus) | [170] |
Azospirillum | Biofertilizer | Barley (Hordeum vulgare), wheat (Triticum aestivum L.), oats | [171] |
Bacillus cereus | Phytoremediation of heavy metals | Vetiveria zizanioides L | [172] |
Bacillus spp. | Auxin synthesis Production of antibiotics, siderophores and enzymes | Potato (Solanum tuberosum), cucumber (Cucumis sativus), peanuts (Arachis hypogaea) | [173] |
Bacillus amyloliquefaciens | Mitigation of nitrous oxide emissions | Acidic soils | [174] |
Burkholderia kururiensis | Nutrients uptake Nitrogen fixation | Sorghum (Sorghum bicolor) | [175] |
Nitrosospira spp. | Management and control of nitrous oxide in soils with excess use of inorganic fertilizers | Tropical soils | [176] |
Pseudomonas putida | Iron translocation through siderophore production | Mung bean (Vigna radiata) | [177] |
Pseudomonas fluorescens | Biocontrol agent against fusarium wilt disease | Tomato (Solanum lycopersicum) | [178] |
Burkholderia paludis | Siderophore and antibiotic production | Forest rhizosphere | [179] |
Bacillus cepacia | Biocontrol agent against fungal pathogens | Pepper plant (Piper nigrum) | [180] |
Rhodobacteria | Nitrogen fixation | Wheat (Triticum aestivum L.) | [181] |
Azarcus | Nitrogen fixation | Rice (Oryza sativa) | [182] |
Azorhizobium | Nitrogen fixation | Sugarcane (Saccharum officinarum) | [183] |
SerratiaMarcescens | Pathogen biocontrol Antifungal | Wheat (Triticum aestivum L.) | [184] |
Pseudomonas aeruginosa | Siderophore production Biocontrol agent against Rhizoctonia solani and Colletotrichum gloeosporioides | Chilli (Capsicum frutescens) | [185] |