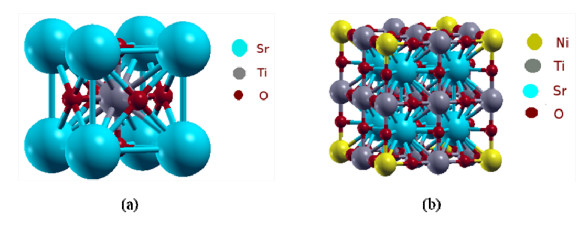
Citation: Mohammad Mofatteh. mRNA localization and local translation in neurons[J]. AIMS Neuroscience, 2020, 7(3): 299-310. doi: 10.3934/Neuroscience.2020016
[1] | Shinichi Tamura, Yoshi Nishitani, Chie Hosokawa . Feasibility of Multiplex Communication in a 2D Mesh Asynchronous Neural Network with Fluctuations. AIMS Neuroscience, 2016, 3(4): 385-397. doi: 10.3934/Neuroscience.2016.4.385 |
[2] | Yoshi Nishitani, Chie Hosokawa, Yuko Mizuno-Matsumoto, Tomomitsu Miyoshi, Shinichi Tamura . Effect of correlating adjacent neurons for identifying communications: Feasibility experiment in a cultured neuronal network. AIMS Neuroscience, 2018, 5(1): 18-31. doi: 10.3934/Neuroscience.2018.1.18 |
[3] | Yang Yu, Tsuyoshi Setogawa, Jumpei Matsumoto, Hiroshi Nishimaru, Hisao Nishijo . Neural basis of topographical disorientation in the primate posterior cingulate gyrus based on a labeled graph. AIMS Neuroscience, 2022, 9(3): 373-394. doi: 10.3934/Neuroscience.2022021 |
[4] | Zinia Pervin, Julia M Stephen . Effect of alcohol on the central nervous system to develop neurological disorder: pathophysiological and lifestyle modulation can be potential therapeutic options for alcohol-induced neurotoxication. AIMS Neuroscience, 2021, 8(3): 390-413. doi: 10.3934/Neuroscience.2021021 |
[5] | Shun Sakuma, Yuko Mizuno-Matsumoto, Yoshi Nishitani, Shinichi Tamura . Learning Times Required to Identify the Stimulated Position and Shortening of Propagation Path by Hebb’s Rule in Neural Network. AIMS Neuroscience, 2017, 4(4): 238-253. doi: 10.3934/Neuroscience.2017.4.238 |
[6] | Yoshi Nishitani, Chie Hosokawa, Yuko Mizuno-Matsumoto, Tomomitsu Miyoshi, Shinichi Tamura . Classification of Spike Wave Propagations in a Cultured Neuronal Network: Investigating a Brain Communication Mechanism. AIMS Neuroscience, 2017, 4(1): 1-13. doi: 10.3934/Neuroscience.2017.1.1 |
[7] | Chacchu Bhattarai, Phanindra Prasad Poudel, Arnab Ghosh, Sneha Guruprasad Kalthur . The RET gene encodes RET protein, which triggers intracellular signaling pathways for enteric neurogenesis, and RET mutation results in Hirschsprung's disease. AIMS Neuroscience, 2022, 9(1): 128-149. doi: 10.3934/Neuroscience.2022008 |
[8] | Yoshi Nishitani, Chie Hosokawa, Yuko Mizuno-Matsumoto, Tomomitsu Miyoshi, Shinichi Tamura . Learning process for identifying different types of communication via repetitive stimulation: feasibility study in a cultured neuronal network. AIMS Neuroscience, 2019, 6(4): 240-249. doi: 10.3934/Neuroscience.2019.4.240 |
[9] | Ernest Greene . New encoding concepts for shape recognition are needed. AIMS Neuroscience, 2018, 5(3): 162-178. doi: 10.3934/Neuroscience.2018.3.162 |
[10] | Jhunlyn Lorenzo, Stéphane Binczak, Sabir Jacquir . A multilayer-multiplexer network processing scheme based on the dendritic integration in a single neuron. AIMS Neuroscience, 2022, 9(1): 76-113. doi: 10.3934/Neuroscience.2022006 |
Photocatalytic water splitting using semiconductor materials provides a way to produce clean hydrogen fuel [1,2,3,4]. A photocatalytic water splitting has to be proper band positions, i.e., the valence band maximum VBM should be more positive than the water oxidation potential and the conduction band minimum CBM must be more negative than the hydrogen reduction potential, the SrTiO3 has benne a most stability and an abundance of raw materials, and thus it is a promising photocatalyst for producing hydrogen from water using solar energy [5,6]. SrTiO3 is a cubic perovskite structure material [7]. However, SrTiO3 use at range of applications in many fields such as, photocatalysis [8,9,10], energy storage [11,12], sensing [13], micro wave devices[14], anode material for lithium-ion batteries [15], H2S solar cells [16], and random access memories[17]. Can be synthesize SrTiO3 by several methods such as sol-gel [18], treatment hydrothermal [8,10,13,19,20], solid state reaction [15], polymeric precursor method [9], pulsed laser decomposition [21,22], co-precipitation/solvo-thermal [15], and electro-spinning [23]. And many studies have been done about metal-doped SrTiO3, such as Fe, La and Mn [24,25,26].
In this work, the structural and electronic properties of both SrTiO3 and SrNi0.125Ti0.875O3 were calculated by using the full potential augmented plane wave plus local orbital (FP-(APW + lo)) method based on density functional theory (DFT) [27]. This is implemented in wien2k code to solve Kohn Sham equation within Full Potential Linearized Augmented Plane Wave (FP-LAPW) method. This method is one of the best methods for appropriate computation of electronic states of various crystalline solids. For structural optimization and the electronic properties the exchange correlation approximation is treated with Perdew-Burke-Ernzerhof Generalized Gradient Approximation (PBE-GGA) Trans-Blaha modified Becke-Johnson (TB-mBJ) potential is employed [28,29,30,31].
The outline of this paper is as follows: in section computational details, we give the computation details. The results and discussion are presented in section results and discussion. Finally section conclusions concludes the paper.
All calculations were performed in the framework of the density function theory (DFT) [27] using the WIEN2k package [32], the full-potential Linearized augmented plane-wave method (FP-LAPW) [27] and the generalized-gradient approximation PBE-GGA with the exchange-correlation functional suggested by Perdew, Burke, and Ernzerhof (PBE) [27].
In these computations, and in order to investigate the structural and electronic properties of SrTiO3 and SrNi0.125Ti0.875O3, the unit cell is separated in two regions, an interstitial region and non-overlapping atomic spheres. In each of these regions, Kohn-Sham wave functions and charge densities as well as electronic potentials are calculated in a different way inside the unit cell. Inside the atomic sphere, they are defined usually by the RMT (radius of muffin tin) around the position of each atom, a spherical harmonic expansion is used, whereas in the interstitial space of the unit cell a plane wave basis set is taken into account. RMT values were chosen as 1.65 a.u. for O, 1.75 a.u. for Ti, 1.8 a.u. for Ni and 2.2 a.u. for Sr. Charge density was Fourier expanded up to Gmax = 12 au−1. The plane wave cut-off Kmax × RMT radius was taken such that RMT × Kmax = 9. For convergence of energy we used a 7 × 7 × 7 k-points grid in the special irreducible Brillouin zone. The self-consistent convergence criteria of total energy calculations of the system were achieved when the total energy stabilized within 10−4 Ry.
For the calculion of structural and electronic properties of Ni doped SrTiO3, we adopt for the SrTiO3 compound—a cubic structure (space group). Pm3m where atomic positions in the elementary cell as presented in Figure 1a are Sr: (0, 0, 0); O: (0, 1/2, 1/2), (1/2, 1/2, 0), (1/2, 0, 1/2); and Ti: (1/2, 1/2, 1/2) When, for the Ni-doped compound SrNi0.125Ti0.875O3, we build a cubic super-cell having twice the cell parameter of the parent SrTiO3 compound Figure 1b whose atomic positions are listed in Table 1.
Atom | Positions |
Sr | (0.75, 0.75, 0.75)(0.25, 0.25, 0.25)(0.25, 0.75, 0.75)(0.75, 0.25, 0.25)(0.25, 0.25, 0.75)(0.75, 0.75, 0.25)(0.75, 0.25, 0.75)(0.25, 0.75, 0.25) |
Ni | (0, 0, 0) |
Ti | (0.5, 0.0, 0.0)(0.0, 0.5, 0.0)(0.0, 0.0, 0.5)(0.0, 0.5, 0.5)(0.5, 0.0, 0.5)(0.5, 0.5, 0.0)(0.5, 0.5, 0.5) |
O | (0.0, 0.0, 0.75)(0.0, 0.0, 0.25)(0.75, 0.0, 0.0)(0.25, 0.0, 0.0)(0.0, 0.75, 0.0)(0.0, 0.25, 0.0)(0.5, 0.0, 0.75) |
All calculations were performed for a 40 atom (5 × 8) super-cell (with a0 = 7.81 Å) (Figure 1b). The optimization of the lattice constants for SrTiO3 and SrNi0.125Ti0.875O3 is made by minimization of the total energy. The calculate lattice parameters, bulk moduli, pressure derivatives B' for SrTiO3 and SrNi0.125Ti0.875O3 were calculated by fitting the total energy E versus volume V data to the non-linear Murnagham equation of state [27]. We present the E (V) relationship of both SrTiO3 and SrNi0.125Ti0.875O3 in Figure 2a, b respectively.
Table 2 presents the calculate lattice parameters, bulk moduli, their pressure derivatives B' together with available experimental data and other theoretical data for both SrTiO3 and SrNi0.125Ti0.875O3. The value of our calculated lattice constants for both SrTiO3 (3.894 Å) and SrNi0.125Ti0.875O3 (3.877 Å). This is in good agreement with the experiment results [7] that the lattice constant of SrNi0.125Ti0.875O3 films increase after doping. From the values of bulk moduli, we can conclude that the SrNi0.125Ti0.875O3 is slightly less stable than SrTiO3.
Compounds | Method | Approximation | Lattice constant (Å) | B (GPa) | B' | Refs. |
SrTiO3 | FP-LAPW | PBE-GGA | 3.894 | 184.489 | 4.41 | Present |
Experimental | Experimental | 3.905 | / | / | [7] | |
FP-LAPW | GGA-96 | 3.905 | 184 | / | [33] | |
3.87 | 194 | / | [34] | |||
SrNi0.125Ti0.875O3 | FP-LAPW | PBE-GGA | 3.877 | 183.62 | 4.44 | Present |
In this section, the electronic structures for both SrTiO3 and SrNi0.125Ti0.875O3 will be studied and compared to each other. Several indicators will be used to detect the effects of Ni doping on the SrTiO3 electronic composition, which is total DOS and states partial density (PDOS). From these tools, some aspects of structural features can be demonstrated. The total DOS and PDOS for SrTiO3 are first calculated for comparison and the results are shown in Figure 3a. For clarity, only the Ti 3d, O 2p and Sr 5p PDOS are shown and this will be evident in the following figures. The top of the valence bands (VBs) mainly consists of O 2p states and the most important vacancy power bands in the lower conduction bands (CBs) mainly consist of Ti 3d states. There is no interference between PDOS between Sr atoms and O atoms, meaning that the Sr-O bonds have a high ion city. Furthermore, the band gap in SrTiO3 is about 2.857 eV, which is less than the experimental value (of about 3.2 eV) [32]. This is naturally underestimated by the DFT [35,36] and by cause the strong self-interaction of the Ti 3d states [37] (Figure 3).The total DOS and PDOS for SrNi0.125Ti0.875O3 are shown in (Figure 3).Where we see the control of both the Ti 3d, Ni 3d, O 2p and Sr 5p PDOS are shown and this will be evident in the following figures. The top of the valence bands (VBs) mainly consists of Ni 3d and O 2p states and the most important vacancy power bands in the lower conduction bands (CBs) mainly consist of Ti 3d states. We also notice no overlap between PDOS Sr atoms and O atoms, meaning that the Sr-O bonds have a high ion. Furthermore, the band gap in SrNi0.125Ti0.875O3 is about 1.078 eV. From all this we conclude that Ni atoms reduce the band gap and improve the effectiveness of SrTiO3.
We calculated the band structures of SrTiO3 (Figure 4), and the effect for all doping by nickel metal atoms on the electronic properties of SrTiO3. We found that the SrTiO3 has an indirect gap Γ-M point of Brillouin zone, and our calculated value of this gap (about 2.857 eV) is lower than the experimental value (around 3.2 eV). The largely, due to the well-known shortcoming of exchange-correction functionals in describing, excited states using the PBE-GGA approximation [38,39]. However, the characters of band structures and the relative variation of band gap are reasonable and reliable, so a scissors worker (1.3 eV) was performed to correct the underestimation of the band gaps of doped SrTiO3 [35,36,37,38,39,40]. Figure 4 shows the calculated band structures SrNi0.125Ti0.875O3 and SrTiO3 around band gap states. Because 1.078 eV, which is an indirect gap (Γ-M point of Brillouin zone). Two isolated defect bands crossing the Brillouin zone make a great decrease to the band gap, thus the optical absorption edge towards the visible light region is largely expanded. The defect bands are close to the valence band, which indicates that Ni dopant atoms are the acceptor impurities, leading to the increase of whole concentration [39].
Figure 5 represents the charge density of SrNi0.125Ti0.875O3 and SrTiO3. The Sr, Ti, and Ni atoms contain more basic electrons, resulting in a higher overall density of charge near the nucleus position, while oxygen atoms are larger with a large number of valence electrons. In the compounds SrTiO3 and SrNi0.125Ti0.875O3, we note a difference in ionic bonds between Ni-O and Ti-O. This is due to the electronic distribution of Ni and Ti atoms.
We investigated the structural and electronic properties of Ni-doped SrTiO3 by a first-principles calculation of FP-LAPW method based on DFT. The calculated results the structure of SrNi0.125Ti0.875O3 is less stable than that of pure SrTiO3. The Ni fully acts as an electron donor in SrNi0.125Ti0.875O3 and the Fermi level shifts into the CBs after Ni doping. The DOS of the SrNi0.125Ti0.875O3 system shift toward low energies and the band gap of SrTiO3 is 2.857 eV and reduced by about 1.779 eV for SrNi0.125Ti0.875O3.
The authors declare that they have no known competing financial interests or personal relationships that could have appeared to influence the work reported in this paper.
[1] |
Mofatteh M, Bullock SL (2017) SnapShot: Subcellular mRNA localization. Cell 169: 178-178.e1. doi: 10.1016/j.cell.2017.03.004
![]() |
[2] |
Holt CE, Schuman EM (2013) The central dogma decentralized: New perspectives on RNA function and local translation in neurons. Neuron 80: 648-657. doi: 10.1016/j.neuron.2013.10.036
![]() |
[3] |
Buxbaum AR, Haimovich G, Singer RH (2015) In the right place at the right time: visualizing and understanding mRNA localization. Nat Rev Mol Cell Biol 16: 95-109. doi: 10.1038/nrm3918
![]() |
[4] |
Jeffery WR, Tomlinson CR, Brodeur RD (1983) Localization of actin messenger RNA during early ascidian development. Dev Biol 99: 408-417. doi: 10.1016/0012-1606(83)90290-7
![]() |
[5] |
Long RM, Singer RH, Meng X, et al. (1997) Mating type switching in yeast controlled by asymmetric localization of ASH1 mRNA. Science 277: 383-387. doi: 10.1126/science.277.5324.383
![]() |
[6] |
Lawrence JB, Singer RH (1986) Intracellular localization of messenger RNAs for cytoskeletal proteins. Cell 45: 407-415. doi: 10.1016/0092-8674(86)90326-0
![]() |
[7] | Tabara H, Hill RJ, Mello CC, et al. (1999) pos-1 encodes a cytoplasmic zinc-finger protein essential for germline specification in C. elegans. Development 126: 1-11. |
[8] |
Berleth T, Burri M, Thoma G, et al. (1988) The role of localization of bicoid RNA in organizing the anterior pattern of the Drosophila embryo. EMBO J 7: 1749-1756. doi: 10.1002/j.1460-2075.1988.tb03004.x
![]() |
[9] |
Melton DA (1987) Translocation of a localized maternal mRNA to the vegetal pole of Xenopus oocytes. Nature 328: 80-82. doi: 10.1038/328080a0
![]() |
[10] |
Houle VM, Li W, Montgomery RK, et al. (2003) mRNA localization in polarized intestinal epithelial cells. Am J Physiol Gastrointest Liver Physiol 284: G722-G727. doi: 10.1152/ajpgi.00458.2002
![]() |
[11] |
Broadus J, Fuerstenberg S, Doe CQ (1998) Staufen-dependent localization of prospero mRNA contributes to neuroblast daughter-cell fate. Nature 391: 792-795. doi: 10.1038/35861
![]() |
[12] |
Garner CC, Tucker RP, Matus A (1988) Selective localization of messenger RNA for cytoskeletal protein MAP2 in dendrites. Nature 336: 674-677. doi: 10.1038/336674a0
![]() |
[13] |
Cajigas IJ, Tushev G, Will TJ, et al. (2012) The local transcriptome in the synaptic neuropil revealed by deep sequencing and high-resolution imaging. Neuron 74: 453-466. doi: 10.1016/j.neuron.2012.02.036
![]() |
[14] |
Köhrmann M, Luo M, Kaether C, et al. (1999) Microtubule-dependent recruitment of Staufen-green fluorescent protein into large RNA-containing granules and subsequent dendritic transport in living hippocampal neurons. Mol Biol Cell 10: 2945-2953. doi: 10.1091/mbc.10.9.2945
![]() |
[15] |
Mikl M, Vendra G, Kiebler MA (2011) Independent localization of MAP2, CaMKIIα and β-actin RNAs in low copy numbers. EMBO Rep 12: 1077-1084. doi: 10.1038/embor.2011.149
![]() |
[16] |
Roos J, Hummel T, Ng N, et al. (2000) Drosophila futsch regulates synaptic microtubule organization and is necessary for synaptic growth. Neuron 26: 371-382. doi: 10.1016/S0896-6273(00)81170-8
![]() |
[17] |
Tiruchinapalli DM, Oleynikov Y, Kelic S, et al. (2003) Activity-dependent trafficking and dynamic localization of zipcode binding protein 1 and beta-actin mRNA in dendrites and spines of hippocampal neurons. J Neurosci 23: 3251-3261. doi: 10.1523/JNEUROSCI.23-08-03251.2003
![]() |
[18] |
Tongiorgi E, Armellin M, Giulianini PG, et al. (2004) Brain-derived neurotrophic factor mRNA and protein are targeted to discrete dendritic laminas by events that trigger epileptogenesis. J Neurosci 24: 6842-6852. doi: 10.1523/JNEUROSCI.5471-03.2004
![]() |
[19] |
Park HY, Lim H, Yoon YJ, et al. (2014) Visualization of dynamics of single endogenous mRNA labeled in live mouse. Science 343: 422-424. doi: 10.1126/science.1239200
![]() |
[20] |
Kanai Y, Dohmae N, Hirokawa N (2004) Kinesin transports RNA: Isolation and characterization of an RNA-Transporting granule. Neuron 43: 513-525. doi: 10.1016/j.neuron.2004.07.022
![]() |
[21] |
Rook MS, Lu M, Kosik KS (2000) CaMKIIalpha 3′ untranslated region-directed mRNA translocation in living neurons: visualization by GFP linkage. J Neurosci 20: 6385-6393. doi: 10.1523/JNEUROSCI.20-17-06385.2000
![]() |
[22] |
Ling SC, Fahrner PS, Greenough WT, et al. (2004) Transport of Drosophila fragile X mental retardation protein-containing ribonucleoprotein granules by kinesin-1 and cytoplasmic dynein. Proc Natl Acad Sci 101: 17428-17433. doi: 10.1073/pnas.0408114101
![]() |
[23] |
Dictenberg JB, Swanger SA, Antar LN, et al. (2008) A direct role for FMRP in activity-dependent dendritic mRNA transport links filopodial-spine morphogenesis to fragile X syndrome. Dev Cell 14: 926-939. doi: 10.1016/j.devcel.2008.04.003
![]() |
[24] |
Kao DI, Aldridge GM, Weiler IJ, et al. (2010) Altered mRNA transport, docking, and protein translation in neurons lacking fragile X mental retardation protein. Proc Natl Acad Sci 107: 15601-15606. doi: 10.1073/pnas.1010564107
![]() |
[25] |
Estes PS, O'Shea M, Clasen S, et al. (2008) Fragile X protein controls the efficacy of mRNA transport in Drosophila neurons. Mol Cell Neurosci 39: 170-179. doi: 10.1016/j.mcn.2008.06.012
![]() |
[26] |
Pan L, Zhang YQ, Woodruff E, et al. (2004) The Drosophila fragile X gene negatively regulates neuronal elaboration and synaptic differentiation. Curr Biol 14: 1863-1870. doi: 10.1016/j.cub.2004.09.085
![]() |
[27] |
Tucker B, Richards RI, Lardelli M (2006) Contribution of mGluR and Fmr1 functional pathways to neurite morphogenesis, craniofacial development and fragile X syndrome. Hum Mol Genet 15: 3446-3458. doi: 10.1093/hmg/ddl422
![]() |
[28] |
Dynes JL, Steward O (2007) Dynamics of bidirectional transport of Arc mRNA in neuronal dendrites. J Comp Neurol 500: 433-447. doi: 10.1002/cne.21189
![]() |
[29] |
Doyle M, Kiebler MA (2011) Mechanisms of dendritic mRNA transport and its role in synaptic tagging. EMBO J 30: 3540-3552. doi: 10.1038/emboj.2011.278
![]() |
[30] |
Wang C, Han B, Zhou R, et al. (2016) Real-time imaging of translation on single mRNA transcripts in live cells. Cell 165: 990-1001. doi: 10.1016/j.cell.2016.04.040
![]() |
[31] |
Weatheritt RJ, Gibson TJ, Babu MM (2014) Asymmetric mRNA localization contributes to fidelity and sensitivity of spatially localized systems. Nat Struct Mol Biol 21: 833-839. doi: 10.1038/nsmb.2876
![]() |
[32] |
Liao G, Mingle L, Van De Water L, et al. (2015) Control of cell migration through mRNA localization and local translation. Wiley Interdiscip Rev RNA 6: 1-15. doi: 10.1002/wrna.1265
![]() |
[33] |
Park HY, Trcek T, Wells AL, et al. (2012) An unbiased analysis method to quantify mRNA localization reveals its correlation with cell motility. Cell Rep 1: 179-184. doi: 10.1016/j.celrep.2011.12.009
![]() |
[34] |
Twiss JL, Fainzilber M (2009) Ribosomes in axons- scrounging from the neighbors? Trends Cell Biol 19: 236-243. doi: 10.1016/j.tcb.2009.02.007
![]() |
[35] |
Eng H, Lund K, Campenot RB (1999) Synthesis of β-Tubulin, actin, and other proteins in axons of sympathetic neurons in compartmented cultures. J Neurosci 19: 1-9. doi: 10.1523/JNEUROSCI.19-01-00001.1999
![]() |
[36] |
Giuditta A, Menichini E, Capano CP, et al. (1991) Active polysomes in the axoplasm of the squid giant axon. J Neurosci Res 28: 18-28. doi: 10.1002/jnr.490280103
![]() |
[37] |
Bassell GJ, Zhang H, Byrd AL, et al. (1998) Sorting of beta-actin mRNA and protein to neurites and growth cones in culture. J Neurosci 18: 251-265. doi: 10.1523/JNEUROSCI.18-01-00251.1998
![]() |
[38] |
Bunge MB (1973) Fine structure of nerve fibers and growth cones of isolated sympathetic neurons in culture. J Cell Biol 56: 713-735. doi: 10.1083/jcb.56.3.713
![]() |
[39] |
Jung H, Yoon BC, Holt CE (2012) Axonal mRNA localization and local protein synthesis in nervous system assembly, maintenance and repair. Nat Rev Neurosci 13: 308-324. doi: 10.1038/nrn3210
![]() |
[40] |
Shigeoka T, Jung H, Jung J, et al. (2016) Dynamic axonal translation in developing and mature visual circuits. Cell 166: 181-192. doi: 10.1016/j.cell.2016.05.029
![]() |
[41] |
Tcherkezian J, Brittis PA, Thomas F, et al. (2010) Transmembrane receptor DCC associates with protein synthesis machinery and regulates translation. Cell 141: 632-644. doi: 10.1016/j.cell.2010.04.008
![]() |
[42] |
Koppers M, Cagnetta R, Shigeoka T, et al. (2019) Receptor-specific interactome as a hub for rapid cue-induced selective translation in axons. eLife 8: e48718. doi: 10.7554/eLife.48718
![]() |
[43] |
Zheng JQ, Kelly TK, Chang B, et al. (2001) A functional role for intra-axonal protein synthesis during axonal regeneration from adult sensory neurons. J Neurosci 21: 9291-9303. doi: 10.1523/JNEUROSCI.21-23-09291.2001
![]() |
[44] |
Merianda TT, Lin AC, Lam JSY, et al. (2009) A functional equivalent of endoplasmic reticulum and Golgi in axons for secretion of locally synthesized proteins. Mol Cell Neurosci 40: 128-142. doi: 10.1016/j.mcn.2008.09.008
![]() |
[45] |
Spencer GE, Syed NI, van Kesteren E, et al. (2000) Synthesis and functional integration of a neurotransmitter receptor in isolated invertebrate axons. J Neurobiol 44: 72-81. doi: 10.1002/1097-4695(200007)44:1<72::AID-NEU7>3.0.CO;2-#
![]() |
[46] |
Cagnetta R, Frese CK, Shigeoka T, et al. (2018) Rapid cue-specific remodeling of the nascent axonal proteome. Neuron 99: 29-46. e4. doi: 10.1016/j.neuron.2018.06.004
![]() |
[47] |
Verma P, Chierzi S, Codd AM, et al. (2005) Axonal protein synthesis and degradation are necessary for efficient growth cone regeneration. J Neurosci 25: 331-342. doi: 10.1523/JNEUROSCI.3073-04.2005
![]() |
[48] |
Campbell DS, Holt CE (2001) Chemotropic responses of retinal growth cones mediated by rapid local protein synthesis and degradation. Neuron 32: 1013-1026. doi: 10.1016/S0896-6273(01)00551-7
![]() |
[49] |
Andreassi C, Zimmermann C, Mitter R, et al. (2010) An NGF-responsive element targets myo-inositol monophosphatase-1 mRNA to sympathetic neuron axons. Nat Neurosci 13: 291-301. doi: 10.1038/nn.2486
![]() |
[50] |
Zivraj KH, Tung YCL, Piper M, et al. (2010) Subcellular profiling reveals distinct and developmentally regulated repertoire of growth cone mRNAs. J Neurosci 30: 15464-15478. doi: 10.1523/JNEUROSCI.1800-10.2010
![]() |
[51] |
Lin AC, Holt CE (2007) Local translation and directional steering in axons. EMBO J 26: 3729-3736. doi: 10.1038/sj.emboj.7601808
![]() |
[52] | Harris WA, Holt CE, Bonhoeffer F (1987) Retinal axons with and without their somata, growing to and arborizing in the tectum of Xenopus embryos: a time-lapse video study of single fibres in vivo. Development 101: 123-133. |
[53] |
Sahoo PK, Smith DS, Perrone-Bizzozero N, et al. (2018) Axonal mRNA transport and translation at a glance. J Cell Sci 131: jcs196808. doi: 10.1242/jcs.196808
![]() |
[54] |
Cosker KE, Fenstermacher SJ, Pazyra-Murphy MF, et al. (2016) The RNA-binding protein SFPQ orchestrates an RNA regulon to promote axon viability. Nat Neurosci 19: 690-696. doi: 10.1038/nn.4280
![]() |
[55] |
Sasaki Y, Welshhans K, Wen Z, et al. (2010) Phosphorylation of zipcode binding protein 1 is required for brain-derived neurotrophic factor signaling of local beta-actin synthesis and growth cone turning. J Neurosci 30: 9349-9358. doi: 10.1523/JNEUROSCI.0499-10.2010
![]() |
[56] |
Spillane M, Ketschek A, Donnelly CJ, et al. (2012) Nerve growth factor-induced formation of axonal filopodia and collateral branches involves the intra-axonal synthesis of regulators of the actin-nucleating Arp2/3 complex. J Neurosci 32: 17671-17689. doi: 10.1523/JNEUROSCI.1079-12.2012
![]() |
[57] |
Wong HHW, Lin JQ, Ströhl F, et al. (2017) RNA docking and local translation regulate site-specific axon remodeling in vivo. Neuron 95: 852-868. e8. doi: 10.1016/j.neuron.2017.07.016
![]() |
[58] |
Mardakheh FK, Paul A, Kümper S, et al. (2015) Global analysis of mRNA, translation, and protein localization: Local translation is a key regulator of cell protrusions. Dev Cell 35: 344-357. doi: 10.1016/j.devcel.2015.10.005
![]() |
[59] |
Shigeoka T, Jung H, Jung J, et al. (2016) Dynamic axonal translation in developing and mature visual circuits. Cell 166: 181-192. doi: 10.1016/j.cell.2016.05.029
![]() |
[60] |
Piper M, Anderson R, Dwivedy A, et al. (2006) Signaling mechanisms underlying Slit2-induced collapse of Xenopus retinal growth cones. Neuron 49: 215-228. doi: 10.1016/j.neuron.2005.12.008
![]() |
[61] |
Meyer MP, Smith SJ (2006) Evidence from in vivo imaging that synaptogenesis guides the growth and branching of axonal arbors by two distinct mechanisms. J Neurosci 26: 3604-3614. doi: 10.1523/JNEUROSCI.0223-06.2006
![]() |
[62] |
Sanchez G, Dury AY, Murray LM, et al. (2012) A novel function for the survival motoneuron protein as a translational regulator. Hum Mol Genet 22: 668-684. doi: 10.1093/hmg/dds474
![]() |
[63] |
Shukla S, Parker R (2016) Hypo- and Hyper-Assembly diseases of RNA-Protein complexes. Trends Mol Med 22: 615-628. doi: 10.1016/j.molmed.2016.05.005
![]() |
[64] |
Jung H, Yoon BC, Holt CE (2012) Axonal mRNA localization and local protein synthesis in nervous system assembly, maintenance and repair. Nat Rev Neurosci 13: 308-324. doi: 10.1038/nrn3210
![]() |
[65] |
Hao le T, Duy PQ, An M, et al. (2017) HuD and the survival motor neuron protein interact in motoneurons and are essential for motoneuron development, function, and mRNA regulation. J Neurosci 37: 11559-11571. doi: 10.1523/JNEUROSCI.1528-17.2017
![]() |
[66] |
Fallini C, Zhang H, Su Y, et al. (2011) The survival of motor neuron (SMN) protein interacts with the mRNA-binding protein HuD and regulates localization of poly(A) mRNA in primary motor neuron axons. J Neurosci 31: 3914-3925. doi: 10.1523/JNEUROSCI.3631-10.2011
![]() |
[67] |
Akten B, Kye MJ, Hao LT, et al. (2011) Interaction of survival of motor neuron (SMN) and HuD proteins with mRNA cpg15 rescues motor neuron axonal deficits. Proc Natl Acad Sci 108: 10337-10342. doi: 10.1073/pnas.1104928108
![]() |
[68] |
Fallini C, Donlin-Asp PG, Rouanet JP, et al. (2016) Deficiency of the survival of motor neuron protein impairs mRNA localization and local translation in the growth cone of motor neurons. J Neurosci 36: 3811-3820. doi: 10.1523/JNEUROSCI.2396-15.2016
![]() |
1. | Yuyoung Joo, David R. Benavides, Local Protein Translation and RNA Processing of Synaptic Proteins in Autism Spectrum Disorder, 2021, 22, 1422-0067, 2811, 10.3390/ijms22062811 | |
2. | Mohammad Mofatteh, Neurosurgery and artificial intelligence, 2021, 8, 2373-7972, 477, 10.3934/Neuroscience.2021025 | |
3. | Lisa Pavinato, Andrea Delle Vedove, Diana Carli, Marta Ferrero, Silvia Carestiato, Jennifer L Howe, Emanuele Agolini, Domenico A Coviello, Ingrid van de Laar, Ping Yee Billie Au, Eleonora Di Gregorio, Alessandra Fabbiani, Susanna Croci, Maria Antonietta Mencarelli, Lucia P Bruno, Alessandra Renieri, Danai Veltra, Christalena Sofocleous, Laurence Faivre, Benoit Mazel, Hana Safraou, Anne-Sophie Denommé-Pichon, Marjon A van Slegtenhorst, Noor Giesbertz, Richard H van Jaarsveld, Anna Childers, R Curtis Rogers, Antonio Novelli, Silvia De Rubeis, Joseph D Buxbaum, Stephen W Scherer, Giovanni Battista Ferrero, Brunhilde Wirth, Alfredo Brusco, CAPRIN1 haploinsufficiency causes a neurodevelopmental disorder with language impairment, ADHD and ASD, 2023, 146, 0006-8950, 534, 10.1093/brain/awac278 | |
4. | Sudhriti Ghosh Dastidar, Deepak Nair, A Ribosomal Perspective on Neuronal Local Protein Synthesis, 2022, 15, 1662-5099, 10.3389/fnmol.2022.823135 | |
5. | Nikoletta Triantopoulou, Marina Vidaki, Local mRNA translation and cytoskeletal reorganization: Mechanisms that tune neuronal responses, 2022, 15, 1662-5099, 10.3389/fnmol.2022.949096 | |
6. | Paola Bonsi, Antonella De Jaco, Laurent Fasano, Paolo Gubellini, Postsynaptic autism spectrum disorder genes and synaptic dysfunction, 2022, 162, 09699961, 105564, 10.1016/j.nbd.2021.105564 | |
7. | Jianqing Wu, John Akighir, FDA Should Re-evaluate All mRNA Vaccines and Revoke Their Use Authorizations (The Short Version)., 2022, 4, 26921537, 16, 10.14302/issn.2692-1537.ijcv-21-4053 | |
8. | Ligia B. Schmitd, Cindy Perez-Pacheco, Emily L. Bellile, Weisheng Wu, Keith Casper, Michelle Mierzwa, Laura S. Rozek, Gregory T. Wolf, Jeremy M.G. Taylor, Nisha J. D'Silva, Spatial and Transcriptomic Analysis of Perineural Invasion in Oral Cancer, 2022, 28, 1078-0432, 3557, 10.1158/1078-0432.CCR-21-4543 | |
9. | Josephine A. Carew, Vivian Cristofaro, Raj K. Goyal, Maryrose P. Sullivan, Differential Myosin 5a splice variants in innervation of pelvic organs, 2023, 14, 1664-042X, 10.3389/fphys.2023.1304537 | |
10. | Mohammad Mofatteh, Mohammad Sadegh Mashayekhi, Saman Arfaie, Yimin Chen, Armaan K. Malhotra, Georgios P. Skandalakis, Mohammed Ali Alvi, Fardad T. Afshari, Shakila Meshkat, Famu Lin, Ebtesam Abdulla, Ayush Anand, Xuxing Liao, Roger S. McIntyre, Carlo Santaguida, Michael H. Weber, Michael G. Fehlings, Anxiety and Depression in Pediatric-Onset Traumatic Spinal Cord Injury: A Systematic Review, 2024, 184, 18788750, 267, 10.1016/j.wneu.2023.12.092 | |
11. | Julia Olivares-Abril, Jana Joha, Jeffrey Y. Lee, Ilan Davis, Optimization of hybridization chain reaction for imaging single RNA molecules in Drosophila larvae , 2024, 18, 1933-6934, 10.1080/19336934.2024.2409968 | |
12. | Wen Xiao, Reem Halabi, Chia-Ho Lin, Mohammad Nazim, Kyu-Hyeon Yeom, Douglas L. Black, The lncRNAMalat1is trafficked to the cytoplasm as a localized mRNA encoding a small peptide in neurons, 2024, 38, 0890-9369, 294, 10.1101/gad.351557.124 | |
13. | Dennis Quentin, Jan S. Schuhmacher, Björn U. Klink, Jeni Lauer, Tanvir R. Shaikh, Pim J. Huis in ’t Veld, Luisa M. Welp, Henning Urlaub, Marino Zerial, Stefan Raunser, Structural basis of mRNA binding by the human FERRY Rab5 effector complex, 2023, 83, 10972765, 1856, 10.1016/j.molcel.2023.05.009 | |
14. | Hikari Nishisaka, Takumi Tomohiro, Kako Fukuzumi, Akira Fukao, Yoshinori Funakami, Toshinobu Fujiwara, Deciphering the Akt1-HuD interaction in HuD-mediated neuronal differentiation, 2024, 221, 03009084, 20, 10.1016/j.biochi.2024.01.010 | |
15. | Kara Snyder, C. Edward Dixon, Jeremy Henchir, Kiersten Gorse, Vincent A. Vagni, Keri Janesko-Feldman, Patrick M. Kochanek, Travis C. Jackson, Gene knockout of RNA binding motif 5 in the brain alters RIMS2 protein homeostasis in the cerebellum and Hippocampus and exacerbates behavioral deficits after a TBI in mice, 2024, 374, 00144886, 114690, 10.1016/j.expneurol.2024.114690 | |
16. | Zhonghao Yu, Yue Guan, Tian Xia, Xuanwen Li, Mingyue Liu, Yujia Huo, Zhuowei Wang, Zhirong Liu, Yuting Luo, Wentao Yan, Lanfang Sun, Wencan Wu, Baoguo Shen, Yikui Zhang, Overcoming Low mRNA Expression in White Matter: A Protocol for RNA Extraction From the Optic Nerve in Large Animals for Transcriptomic Analysis, 2024, 65, 1552-5783, 25, 10.1167/iovs.65.13.25 | |
17. | Mohammad Mofatteh, Examining the association between traumatic brain injury and headache, 2021, 20, 0219-6352, 10.31083/j.jin2004109 | |
18. | Pargol Tayefeh Ghahremani, Soha BaniArdalan, Parsa Alehossein, Arshi Parveen, Masoumeh Jorjani, Candice M. Brown, Werner J. Geldenhuys, Jason D. Huber, Tauheed Ishrat, Sanaz Nasoohi, Poststroke hyperglycemia dysregulates cap-dependent translation in neural cells, 2025, 361, 00243205, 123336, 10.1016/j.lfs.2024.123336 | |
19. | Alexandria L. Quillin, Diane B. Karloff, Tewoderos M. Ayele, Tatiana F. Flores, Gerry Chen, Zachary T. McEachin, Arielle N. Valdez-Sinon, Jennifer M. Heemstra, Imaging and Tracking RNA in Live Mammalian Cells via Fluorogenic Photoaffinity Labeling, 2025, 1554-8929, 10.1021/acschembio.4c00848 | |
20. | Vanessa Lakis, Noni L Chan, Ruth Lyons, Nicola Blackburn, Tam Hong Nguyen, Crystal Chang, Andrew Masel, Nicholas P. West, Glen M. Boyle, Ann-Marie Patch, Anthony J. Gill, Katia Nones, Spatial Transcriptomics Reveals Novel Mechanisms Involved in Perineural Invasion in Pancreatic Ductal Adenocarcinomas, 2025, 17, 2072-6694, 852, 10.3390/cancers17050852 | |
21. | David Zimmermann, Michaela Kress, Istvan Nagy, Established and emerging roles of protein kinases in regulating primary sensory neurons in injury-and inflammation-associated pain, 2025, 1472-8222, 10.1080/14728222.2025.2489540 |
Atom | Positions |
Sr | (0.75, 0.75, 0.75)(0.25, 0.25, 0.25)(0.25, 0.75, 0.75)(0.75, 0.25, 0.25)(0.25, 0.25, 0.75)(0.75, 0.75, 0.25)(0.75, 0.25, 0.75)(0.25, 0.75, 0.25) |
Ni | (0, 0, 0) |
Ti | (0.5, 0.0, 0.0)(0.0, 0.5, 0.0)(0.0, 0.0, 0.5)(0.0, 0.5, 0.5)(0.5, 0.0, 0.5)(0.5, 0.5, 0.0)(0.5, 0.5, 0.5) |
O | (0.0, 0.0, 0.75)(0.0, 0.0, 0.25)(0.75, 0.0, 0.0)(0.25, 0.0, 0.0)(0.0, 0.75, 0.0)(0.0, 0.25, 0.0)(0.5, 0.0, 0.75) |
Compounds | Method | Approximation | Lattice constant (Å) | B (GPa) | B' | Refs. |
SrTiO3 | FP-LAPW | PBE-GGA | 3.894 | 184.489 | 4.41 | Present |
Experimental | Experimental | 3.905 | / | / | [7] | |
FP-LAPW | GGA-96 | 3.905 | 184 | / | [33] | |
3.87 | 194 | / | [34] | |||
SrNi0.125Ti0.875O3 | FP-LAPW | PBE-GGA | 3.877 | 183.62 | 4.44 | Present |
Atom | Positions |
Sr | (0.75, 0.75, 0.75)(0.25, 0.25, 0.25)(0.25, 0.75, 0.75)(0.75, 0.25, 0.25)(0.25, 0.25, 0.75)(0.75, 0.75, 0.25)(0.75, 0.25, 0.75)(0.25, 0.75, 0.25) |
Ni | (0, 0, 0) |
Ti | (0.5, 0.0, 0.0)(0.0, 0.5, 0.0)(0.0, 0.0, 0.5)(0.0, 0.5, 0.5)(0.5, 0.0, 0.5)(0.5, 0.5, 0.0)(0.5, 0.5, 0.5) |
O | (0.0, 0.0, 0.75)(0.0, 0.0, 0.25)(0.75, 0.0, 0.0)(0.25, 0.0, 0.0)(0.0, 0.75, 0.0)(0.0, 0.25, 0.0)(0.5, 0.0, 0.75) |
Compounds | Method | Approximation | Lattice constant (Å) | B (GPa) | B' | Refs. |
SrTiO3 | FP-LAPW | PBE-GGA | 3.894 | 184.489 | 4.41 | Present |
Experimental | Experimental | 3.905 | / | / | [7] | |
FP-LAPW | GGA-96 | 3.905 | 184 | / | [33] | |
3.87 | 194 | / | [34] | |||
SrNi0.125Ti0.875O3 | FP-LAPW | PBE-GGA | 3.877 | 183.62 | 4.44 | Present |