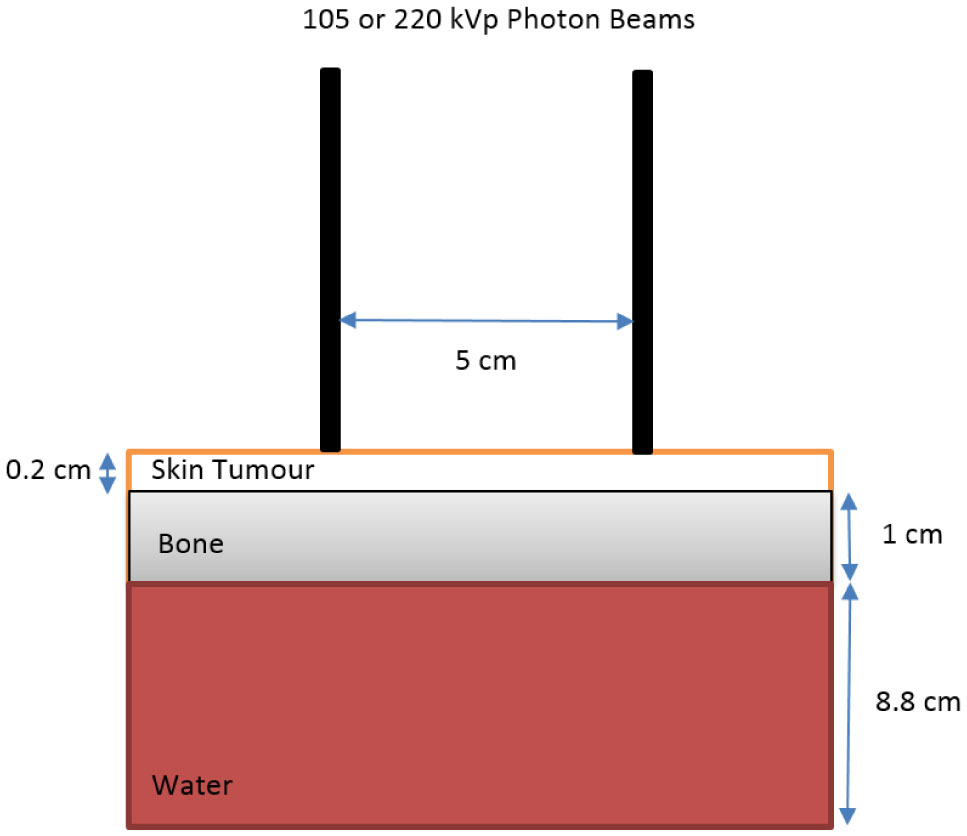
This study compared the dose enhancement predicted in kilovoltage gold nanoparticle-enhanced radiotherapy using the newly developed EGS lattice and the typical gold-water mixture method in Monte Carlo simulation. This new method considered the gold nanoparticle-added volume consisting of solid nanoparticles instead of a gold-water mixture. In addition, this particle method is more realistic in simulation.
A heterogeneous phantom containing bone and water was irradiated by the 105 and 220 kVp x-ray beams. Gold nanoparticles were added to the tumour volume with concentration varying from 3–40 mg/mL in the phantom. The dose enhancement ratio (DER), defined as the ratio of dose at the tumour with and without adding gold nanoparticles, was calculated by the gold-water mixture and particle method using Monte Carlo simulation for comparison.
It is found that the DER was 1.44–4.71 (105 kVp) and 1.27–2.43 (220 kVp) for the gold nanoparticle concentration range of 3–40 mg/mL, when they were calculated by the gold-water mixture method. The DER was slightly larger and equal to 1.47–4.84 (105 kVp) and 1.29–2.5 (220 kVp) for the same concentration range, when the particle method was used. Moreover, the DER predicted by both methods increased with an increase of nanoparticle concentration, and a decrease of x-ray beam energy.
The deviation of DER determined by the particle and gold-water mixture method was insignificant when considering the uncertainty in the calculation of DER (2%) in the nanoparticle concentration range of 3–40 mg/mL. It is therefore concluded that the gold-water mixture method could predict the dose enhancement as accurate as the newly developed particle method.
Citation: Zaynah Sheeraz, James C.L. Chow. Evaluation of dose enhancement with gold nanoparticles in kilovoltage radiotherapy using the new EGS geometry library in Monte Carlo simulation[J]. AIMS Biophysics, 2021, 8(4): 337-345. doi: 10.3934/biophy.2021027
This study compared the dose enhancement predicted in kilovoltage gold nanoparticle-enhanced radiotherapy using the newly developed EGS lattice and the typical gold-water mixture method in Monte Carlo simulation. This new method considered the gold nanoparticle-added volume consisting of solid nanoparticles instead of a gold-water mixture. In addition, this particle method is more realistic in simulation.
A heterogeneous phantom containing bone and water was irradiated by the 105 and 220 kVp x-ray beams. Gold nanoparticles were added to the tumour volume with concentration varying from 3–40 mg/mL in the phantom. The dose enhancement ratio (DER), defined as the ratio of dose at the tumour with and without adding gold nanoparticles, was calculated by the gold-water mixture and particle method using Monte Carlo simulation for comparison.
It is found that the DER was 1.44–4.71 (105 kVp) and 1.27–2.43 (220 kVp) for the gold nanoparticle concentration range of 3–40 mg/mL, when they were calculated by the gold-water mixture method. The DER was slightly larger and equal to 1.47–4.84 (105 kVp) and 1.29–2.5 (220 kVp) for the same concentration range, when the particle method was used. Moreover, the DER predicted by both methods increased with an increase of nanoparticle concentration, and a decrease of x-ray beam energy.
The deviation of DER determined by the particle and gold-water mixture method was insignificant when considering the uncertainty in the calculation of DER (2%) in the nanoparticle concentration range of 3–40 mg/mL. It is therefore concluded that the gold-water mixture method could predict the dose enhancement as accurate as the newly developed particle method.
In radiotherapy, ionizing radiation such as photon or electron is used to irradiate the tumour or target inside the patient to provide cancer control. A radiation treatment plan is therefore created by the radiotherapist, medical physicist and radiation oncologist, focusing on delivering high radiation dose to the tumour, while at the same time limiting the dose received by the surrounding normal tissues or critical organs [1]. To achieve this aim, different dose delivery techniques such as intensity modulated radiotherapy and volumetric modulated arc therapy are used, taking advantage of recent advances of multileaf collimator and inverse treatment planning [2]–[4].
To date, radiosensitizers such as gold nanoparticles are used in radiotherapy to enhance the treatment outcome [5]–[7]. Gold nanoparticle is uptaken by the cancer cell and has some unique qualities that make it a very effective radiosensitizer used in radiotherapy. Gold nanoparticles can enhance the radiation effect in a vast area without being delivered to all tumour cells [8],[9]. As gold nanoparticles are easily absorbed in the circulatory system, they can easily permeate the tumour, resulting in a higher retention effect [10]. Gold nanoparticles are also preferred as they are easily manipulated; their size and shape can be changed easily, and hence they can be customized for different tumour sizes for maximum effect [11],[12]. In radiotherapy, gold nanoparticles can be highly localized to just the tumour cells.
Gold nanoparticle addition to tumour can lead to the following effects on the cancer cell kill. First, the addition of gold nanoparticles to the cancer cell increases the compositional atomic number of the tumour. This increases the absorbed dose at the tumour due to the photoelectric enhancement, and such effect is more significant when kilovoltage (kV) x-ray beams are used in irradiation [13],[14]. Second, the increase of absorbed dose in the target compared to the surrounding tissues results in a larger image contrast of tumour in computed tomography [15],[16]. This can help the radiation oncologist to visualize and highlight the tumour easily in radiation treatment planning. Moreover, it can increase the patient setup accuracy using kV cone-beam computed tomography in image-guided radiotherapy. Currently, there are many preclinical studies and clinical trials being conducted in gold nanoparticle-enhanced radiotherapy, focusing on various aspects in treatment delivery such as radiation dosimetry [17]–[20].
One important study in using gold nanoparticles as radiosensitizer in radiotherapy is to determine the dose enhancement with addition of nanoparticles [21]–[23]. In radiotherapy, dose enhancement ratio (DER), defined as the dose ratio of the target/tumour with and without addition of gold nanoparticles, is a popular dosimetric parameter showing how effective the increase of dose is, when nanoparticles are added to the target [24]. To determine the dose at the tumour, Monte Carlo simulation plays a very important role in predicting the energy deposition at a volume-of-interest (tumour) due to particle interactions from the radiation beam and irradiated medium. Monte Carlo simulation is the benchmark of dose calculation in radiation treatment planning to predict dosimetry in a heterogeneous system with tissue, air and bone [25]. The disadvantage of Monte Carlo method is its relatively longer computing time. However, with the recent rapid development of the computer processor, the calculation speed has improved a lot, reaching an acceptable level from days to hours [26].
There are two approaches to determine the dosimetry in gold nanoparticle-enhanced radiotherapy using Monte Carlo simulation. One is to focus on objects in nanometer scale such as a single gold nanoparticle and a deoxyribonucleic acid (DNA) molecule [27]. In this case, Monte Carlo simulations modelling particle interactions at the nanoscale level in the energy range down to few eV [28],[29]. Monte Carlo codes such as Geant4-DNA are used to study the effect of radiation at the nanometer scale [30]. However, to study the dose enhancement of a tumour with gold nanoparticle addition in a patient, a macroscopic approach is used because of the physical size of tumour ranging from millimeter to centimeter scale. In the millimeter scale, it is very difficult to consider nanoparticles as unique solid particles, because there are a huge number of nanoparticles distributed inside the tumour volume. In this event, the tumour with the addition of gold nanoparticles is considered as a gold and soft tissue (or water) mixture in Monte Carlo simulation [24]. This assumption works well and is used by some studies, showing that gold nanoparticles improved the DER of the tumour, making it radiosensitized, and therefore increased the cancer cell kill in radiotherapy [31]–[33]. Most of these studies used Monte Carlo simulation (EGSnrc code) imitating clinical settings [34]. Recently, a newly developed EGS_lattice geometry was added to the EGSnrc, egs++ source code [35]. This new library allows gold nanoparticle to be directly inserted into the tumour without being mixed with water or tissue.
This study investigated whether using the EGS_lattice geometry (particle method) would vary the Monte Carlo results of DER, when compared to the typical gold-water mixture method. A Monte Carlo phantom study using kV x-ray beams was conducted to compare the DER with gold nanoparticle addition, based on the particle and gold-water mixture method.
A heterogeneous phantom was used in this study as shown in Figure 1. The dimension of the phantom was 10 × 10 × 10 cm3. The phantom was 10 cm depth, consisting of 3 layers, namely, water/water with gold (skin tumour), bone and water, with depths (from the top to bottom) equal to 0.2 cm, 1 cm and 8.8 cm, respectively. This phantom was to mimic the geometry of a skin tumour located in the soft tissue with 0.2 cm thickness on top of a bone (e.g. human forehead). Gold nanoparticles could be transported to the skin tumour in the top layer. This changes the composition of the layer, to a layer containing both water and gold nanoparticles with different concentrations. The middle layer consisted of a bone, and the bottom layer consisted of water. The bone was made up of the ICRU material composed of 10.1% hydrogen, 11.1% carbon, 2.6% nitrogen, and 76.2% oxygen [36]. The 105 and 220 kVp x-ray beams were applied from the top. The gold nanoparticles were only added to the top layer with concentration changed.
The Monte Carlo simulation in this study was based on the EGSnrc code, which was acquired and downloaded for free from GitHub [37]. The kV x-ray beam model was based on the phase-space files of the 105 (HVL: 0.19 mm Al) and 220 kVp (HVL: 0.02 mm Cu) x-ray beams produced by a Gulmay D3225 orthovoltage treatment unit using a standard open circular applicator with diameter equal to 5 cm [32]. The phase-space files were generated by the EGSnrc-based BEAMnrc code with the source-to-surface distance equal to 20 cm [38]. The scoring plane of the phase-space file was at the surface of the phantom. The energy cut-off for the electron (ECUT) and photon (PCUT) transport were set to 521 keV and 1 keV. The dose in the skin tumour layer was determined using the DOSXYZnrc code by setting the source type equal to 2 (isource = 2), for a phase-space source with particles incident from any direction.
For the skin tumour with addition of gold nanoparticles, the composition of the top layer in Figure 1 was modelled by the gold-water mixture and particle method. In this study, different gold nanoparticle concentrations, namely, 3, 7, 18, 30 and 40 mg/mL were used in simulations. This concentration range was set by considering Hainfeld et al [39]. The physical densities of the mixture with concentrations equal to 3, 7, 18, 30 and 40 mg/mL were calculated to be 1.003, 1.007, 1.018, 1.03 and 1.04 g cm−3, respectively. For the gold-water mixture method, related material data set regarding the specific gold nanoparticle concentrations were created using the EGSnrc-based PEGS4 code [40]. The medium composition of the skin tumour (water) added with gold nanoparticles (gold) was calculated and input to the PEGS4 code as per the nanoparticle concentration. Data sets of particle interaction cross-sections for different gold nanoparticle concentrations in the gold-water mixture were generated. For the particle method, the recently developed EGS_lattice library for gold nanoparticle simulation was used [35]. The base code was used to create an input file to run the simulation using egs++. The data used in the PEGS4 data file provided media definition. The nanoparticle size was 100 nm in diameter. These nanoparticles were packed as hexagonal lattice layers of spheres in water [35]. The DOSXYZnrc code was used to determine the dose deposited in the layer with gold nanoparticles [41]. Both simulations using the gold-water mixture and particle method were run twice, once with a 105 kVp x-ray beam and once with a 220 kVp x-ray beam. The results were used to calculate the DER and then compared. It is hypothesized that the new particle method using the EGS_lattice geometry will be a more realistic method to determine the DER, and provide more accurate dose enhancement information in comparison to the gold-water mixture method [35].
The DER reflecting the dose enhancement of the tumour with addition of gold nanoparticles was calculated by the doses at the skin target layer with different gold nanoparticle concentrations. The DER is defined as [32]:
where D Gold Nanoparticles is the dose of the target layer with addition of gold nanoparticles, and Do is the dose of the same layer without addition of gold nanoparticles. When there is no gold nanoparticle added to the skin tumour, the DER is equal to one.
Figure 2 shows the DER of surface tumour layer with different gold nanoparticle concentrations using the gold-water mixture and particle method. The DER using the 105 and 220 kVp x-ray beams are shown in Figure 2(a) and 2(b), respectively. DER value larger than one demonstrated that dose enhancement was present when nanoparticles were added to the skin tumour irradiated by the kV x-ray beams. The uncertainty of the DER is 2% based on the calculated dose uncertainty of 1%.
In Figure 2, it is seen that the DER increased with an increase of gold nanoparticle concentration. For the 105 kVp x-ray beam with concentration range of 3–40 mg/mL, the rate of increase of DER was 0.088 per mL calculated by the gold-water mixture method. The rate of increase of DER was equal to 0.091 per mL when calculated by the particle method. For the 220 kVp x-ray beam, the rate of increase of DER was 0.031 and 0.033 per mL calculated by the gold-water mixture and particle method, respectively. The rate of increase of DER per mL was found higher in the low-energy x-ray beam (105 kVp). This is because of the larger photoelectric cross-section of the low-energy x-ray beam in the kV range [14]. This results in a larger yield of photoelectric electrons (secondary electrons) and therefore higher dose enhancement [8]. When low-energy beam was used, the increase of DER was in the range of 13–94% (gold-water mixture method) and 14–94% (particle method), as the gold nanoparticle concentration increased from 3 to 40 mg/mL. It is seen that a larger increase of DER occurred with a decrease of x-ray beam energy, when a higher gold nanoparticle concentration was used.
Figure 2 shows the relationship between the DER and gold nanoparticle concentration using the gold-water mixture and particle method. The DER was equal to 1.44–4.71 and 1.27–2.43 in the concentration range of 3–40 mg/mL, and for the 105 and 220 kVp x-ray beams using the gold-water mixture method. When the particle method was used, the DER was equal to 1.47–4.84 and 1.29–2.50 in the same concentration range and using the 105 and 220 kVp x-ray beam energy. In the figure, it is seen that a larger DER was predicted, when using the particle method in Monte Carlo simulation. For the 105 kVp x-ray beam, the average increase of DER was equal to 2.1–2.8% in the concentration range of 3–40 mg/mL, when the particle method was used compared to the gold-water mixture method. Similar increase of DER (1.6–2.9%) was predicted using the particle method for the 220 kVp x-ray beam, with the same nanoparticle concentration range. It should be noted that this increase of DER is between the mean DER values estimated with the Monte Carlo simulation. However, it is seen that the deviation between the gold-water mixture and particle method was slightly larger when higher nanoparticle concentration and high-energy x-ray beam (220 kVp) were used. Assuming the particle method can create a better nanoparticle model in the simulation, the gold-water mixture method is found very close to the particle method in this study.
This study compared the DER calculated using the newly developed particle method (based on EGSnrc_lattice library) and the general gold-water mixture method in macroscopic Monte Carlo simulation. Based on a heterogeneous phantom, with different gold nanoparticle concentrations irradiated by kV x-ray beams with different energies, it is found that both methods predicted an increase of DER with an increase of gold nanoparticle concentration and a decrease of x-ray beam energy. Moreover, the deviation of DER derived from the particle and gold-mixture method is found to be insignificant in the nanoparticle concentration range of 3–40 mg/mL. Based on a more realistic model considering lattice of solid gold nanoparticles in the volume, it is concluded that the calculation of DER would be reasonably accurate when considering the gold nanoparticle-added volume as a gold-water mixture.
[1] | Khan FM, Gibbons JP, Sperduto PW (2016) Khan's Treatment Planning in Radiation Oncology Wolters Kluwer. |
[2] |
Antoine M, Ralite F, Soustiel C, et al. (2019) Use of metrics to quantify IMRT and VMAT treatment plan complexity: A systematic review and perspectives. Phys Med 64: 98-108. doi: 10.1016/j.ejmp.2019.05.024
![]() |
[3] |
Bortfeld T (2006) IMRT: a review and preview. Phys Med Biol 51: R363-379. doi: 10.1088/0031-9155/51/13/R21
![]() |
[4] |
Staffurth J (2010) A review of the clinical evidence for intensity-modulated radiotherapy. Clin Oncol 22: 643-657. doi: 10.1016/j.clon.2010.06.013
![]() |
[5] |
Penninckx S, Heuskin AC, Michiels C, et al. (2020) Gold nanoparticles as a potent radiosensitizer: A transdisciplinary approach from physics to patient. Cancers 12: 2021. doi: 10.3390/cancers12082021
![]() |
[6] |
Siddique S, Chow JCL (2020) Gold nanoparticles for drug delivery and cancer therapy. App Sci 10: 3824. doi: 10.3390/app10113824
![]() |
[7] |
Moore JA, Chow JCL (2021) Recent progress and applications of gold nanotechnology in medical biophysics using artificial intelligence and mathematical modeling. Nano Ex 2: 022001. doi: 10.1088/2632-959X/abddd3
![]() |
[8] | Abolfazli MK, Mahdavi SR, Ataei G (2015) Studying effects of gold nanoparticle on dose enhancement in megavoltage radiation. J Biomed Phys Eng 5: 185-190. |
[9] |
Kuncic Z, Lacombe S (2018) Nanoparticle radio-enhancement: principles, progress and application to cancer treatment. Phys Med Biol 63: 02TR01. doi: 10.1088/1361-6560/aa99ce
![]() |
[10] |
Li W, Zhao X, Du B, et al. (2016) Gold nanoparticle–mediated targeted delivery of recombinant human endostatin normalizes tumour vasculature and improves cancer therapy. Sci Rep 6: 1-11. doi: 10.1038/s41598-016-0001-8
![]() |
[11] |
Grzelczak M, Pérez-Juste J, Mulvaney P, et al. (2008) Shape control in gold nanoparticle synthesis. Chem Soc Rev 37: 1783-1791. doi: 10.1039/b711490g
![]() |
[12] |
Liu XY, Wang JQ, Ashby CR, et al. (2021) Gold nanoparticles: synthesis, physiochemical properties and therapeutic applications in cancer. Drug Discov Today 26: 1284-1292. doi: 10.1016/j.drudis.2021.01.030
![]() |
[13] |
Engels E, Bakr S, Bolst D, et al. (2020) Advances in modelling gold nanoparticle radiosensitization using new Geant4-DNA physics models. Phys Med Biol 65: 225017. doi: 10.1088/1361-6560/abb7c2
![]() |
[14] |
Torrisi L (2021) Physical aspects of gold nanoparticles as cancer killer therapy. Ind J Phys 95: 225-234. doi: 10.1007/s12648-019-01679-1
![]() |
[15] |
Ahn S, Jung SY, Lee SJ (2013) Gold nanoparticle contrast agents in advanced X-ray imaging technologies. Molecules 18: 5858-5890. doi: 10.3390/molecules18055858
![]() |
[16] |
Dong YC, Hajfathalian M, Maidment PSN, et al. (2019) Effect of gold nanoparticle size on their properties as contrast agents for computed tomography. Sci Rep 9: 14912. doi: 10.1038/s41598-019-50332-8
![]() |
[17] |
Jain S, Hirst DG, O'sullivan JM (2012) Gold nanoparticles as novel agents for cancer therapy. Brit J Radiol 85: 101-113. doi: 10.1259/bjr/59448833
![]() |
[18] |
Bobo D, Robinson KJ, Islam J, et al. (2016) Nanoparticle-based medicines: a review of FDA-approved materials and clinical trials to date. Pharm Res 33: 2373-2387. doi: 10.1007/s11095-016-1958-5
![]() |
[19] |
Siddique S, Chow JCL (2020) Application of nanomaterials in biomedical imaging and cancer therapy. Nanomaterials 10: 1700. doi: 10.3390/nano10091700
![]() |
[20] |
Butterworth KT, Nicol JR, Ghita M, et al. (2016) Preclinical evaluation of gold-DTDTPA nanoparticles as theranostic agents in prostate cancer radiotherapy. Nanomedicine 11: 2035-2047. doi: 10.2217/nnm-2016-0062
![]() |
[21] |
Chow JCL (2020) Depth dose enhancement on flattening-filter-free X-ray beam: A Monte Carlo study in nanoparticle-enhanced radiotherapy. App Sci 10: 7052. doi: 10.3390/app10207052
![]() |
[22] |
Kakade NR, Sharma SD (2015) Dose enhancement in gold nanoparticle-aided radiotherapy for the therapeutic X-ray beams using Monte Carlo technique. J Cancer Res Ther 11: 94. doi: 10.4103/0973-1482.147691
![]() |
[23] |
Jones BL, Krishnan S, Cho SH (2010) Estimation of microscopic dose enhancement factor around gold nanoparticles by Monte Carlo calculations. Med Phys 37: 3809-3816. doi: 10.1118/1.3455703
![]() |
[24] |
Cho SH (2005) Estimation of tumour dose enhancement due to gold nanoparticles during typical radiation treatments: a preliminary Monte Carlo study. Phys Med Biol 50: N163. doi: 10.1088/0031-9155/50/15/N01
![]() |
[25] |
Rogers DWO (2006) Fifty years of Monte Carlo simulations for medical physics. Phys Med Biol 51: R287. doi: 10.1088/0031-9155/51/13/R17
![]() |
[26] |
Miras H, Jiménez R, Miras C, et al. (2013) CloudMC: a cloud computing application for Monte Carlo simulation. Phys Med Biol 58: N125-N133. doi: 10.1088/0031-9155/58/8/N125
![]() |
[27] |
Chun H, Chow JCL (2016) Gold nanoparticle DNA damage in radiotherapy: A Monte Carlo study. AIMS Bioeng 3: 352-361. doi: 10.3934/bioeng.2016.3.352
![]() |
[28] |
Poignant F, Monini C, Testa É, et al. (2021) Influence of gold nanoparticles embedded in water on nanodosimetry for keV photon irradiation. Med Phys 48: 1874-1883. doi: 10.1002/mp.14576
![]() |
[29] |
Chow JCL (2018) Recent progress in Monte Carlo simulation on gold nanoparticle radiosensitization. AIMS Biophys 5: 231-244. doi: 10.3934/biophy.2018.4.231
![]() |
[30] |
Incerti S, Baldacchino G, Bernal M, et al. (2010) The geant4-dna project. Int J Model Simul Sci Comp 1: 157-178. doi: 10.1142/S1793962310000122
![]() |
[31] |
Martelli S, Chow JCL (2020) Dose enhancement for the flattening-filter-free and flattening-filter X-ray beams in nanoparticle-enhanced radiotherapy: A Monte Carlo phantom study. Nanomaterials 10: 637. doi: 10.3390/nano10040637
![]() |
[32] |
Zheng XJ, Chow JCL (2017) Radiation dose enhancement in skin therapy with nanoparticle addition: A Monte Carlo study on kilovoltage photon and megavoltage electron beams. World J Radiol 9: 63-71. doi: 10.4329/wjr.v9.i2.63
![]() |
[33] |
Koger B, Kirkby C (2016) A method for converting dose-to-medium to dose-to-tissue in Monte Carlo studies of gold nanoparticle-enhanced radiotherapy. Phys Med Biol 61: 2014. doi: 10.1088/0031-9155/61/5/2014
![]() |
[34] |
Kawrakow I (2000) Accurate condensed history Monte Carlo simulation of electron transport. I. EGSnrc, the new EGS4 version. Med Phys 27: 485-498. doi: 10.1118/1.598917
![]() |
[35] |
Martinov MP, Thomson RM (2020) Taking EGSnrc to new lows: Development of egs++ lattice geometry and testing with microscopic geometries. Med Phys 47: 3225-3232. doi: 10.1002/mp.14172
![]() |
[36] | International ommission on Radiation Units and Measurements Tissue substitutes in radiation dosimetry and measurements, United States (1989) . |
[37] | Kawrakow I (2005) egspp: the EGSnrc C++ class library. Technical Report, PIRS-899 National Research Council Canada: Ottawa, Canada. |
[38] | Rogers DWO, Walters B, Kawrakow I (2009) BEAMnrc users manual. NRC Report PIRS 509: 12. |
[39] |
Hainfeld JF, Slatkin DN, Smilowitz HM (2004) The use of gold nanoparticles to enhance radiotherapy in mice. Phys Med Biol 49: N309. doi: 10.1088/0031-9155/49/18/N03
![]() |
[40] | Rogers DWO, Kawrakow I, Seuntjens JP, et al. (2003) NRC user codes for EGSnrc: NRC Technical Report PIRS 702 (rev C) National Research Council Canada: Ottawa, Canada. |
[41] | Walters BR, Kawrakow I, Rogers DWO (2005) DOSXYZnrc users manual. NRC Report PIRS 794: 57-58. |
1. | Afia Sadiq, James C. L. Chow, Evaluation of Dosimetric Effect of Bone Scatter on Nanoparticle-Enhanced Orthovoltage Radiotherapy: A Monte Carlo Phantom Study, 2022, 12, 2079-4991, 2991, 10.3390/nano12172991 | |
2. | Armando Spina, James C. L. Chow, Dosimetric Impact on the Flattening Filter and Addition of Gold Nanoparticles in Radiotherapy: A Monte Carlo Study on Depth Dose Using the 6 and 10 MV FFF Photon Beams, 2022, 15, 1996-1944, 7194, 10.3390/ma15207194 | |
3. | James Chun Lam Chow, 2022, 9780128228197, 291, 10.1016/B978-0-12-822819-7.00002-8 | |
4. | Mirko Salomón Alva-Sánchez, Ney Souza Neto, Ana Quevedo, Relative dose-response from solid-state and gel dosimeters through Monte Carlo simulations, 2022, 10, 2319-0612, 10.15392/bjrs.v10i3.2049 | |
5. | James C.L. Chow, 2024, 9780443216688, 371, 10.1016/B978-0-443-21668-8.00013-4 | |
6. | James C. L. Chow, 2024, Chapter 7, 978-981-97-0220-6, 177, 10.1007/978-981-97-0221-3_7 | |
7. | Wafa M. Al-Saleh, Turki Almatani, Dose enhancement of nanogold for prostate radiation: Monte Carlo simulation using the ICRP voxel computational male phantom, 2024, 18, 1658-3655, 10.1080/16583655.2024.2340138 | |
8. | Elham Mansouri, Saeed Rajabpour, Asghar Mesbahi, In silico estimation of polyethylene glycol coating effect on metallic NPs radio-sensitization in kilovoltage energy beams, 2024, 18, 2661-801X, 10.1186/s13065-024-01322-z | |
9. | Turki Almatani, Wafa M. Al-Saleh, Monte Carlo study on nanogold dose enhancement for breast radiotherapy using an ICRP female computational phantom, 2024, 18, 1658-3655, 10.1080/16583655.2024.2319367 | |
10. | Sandun Jayarathna, Amrit Kaphle, Sunil Krishnan, Sang Hyun Cho, Nanoscale gold nanoparticle (GNP)‐laden tumor cell model and its use for estimation of intracellular dose from GNP‐induced secondary electrons, 2024, 51, 0094-2405, 6276, 10.1002/mp.17275 | |
11. | Adil Bardane, Nabil Maalej, El Mahjoub Chakir, El Mehdi Al Ibrahmi, Gold nanoparticle effect on dose and DNA damage enhancement in the vicinity of gold nanoparticles, 2024, 3, 27731839, 100126, 10.1016/j.nucana.2024.100126 | |
12. | James C. L. Chow, Monte Carlo Simulations in Nanomedicine: Advancing Cancer Imaging and Therapy, 2025, 15, 2079-4991, 117, 10.3390/nano15020117 |