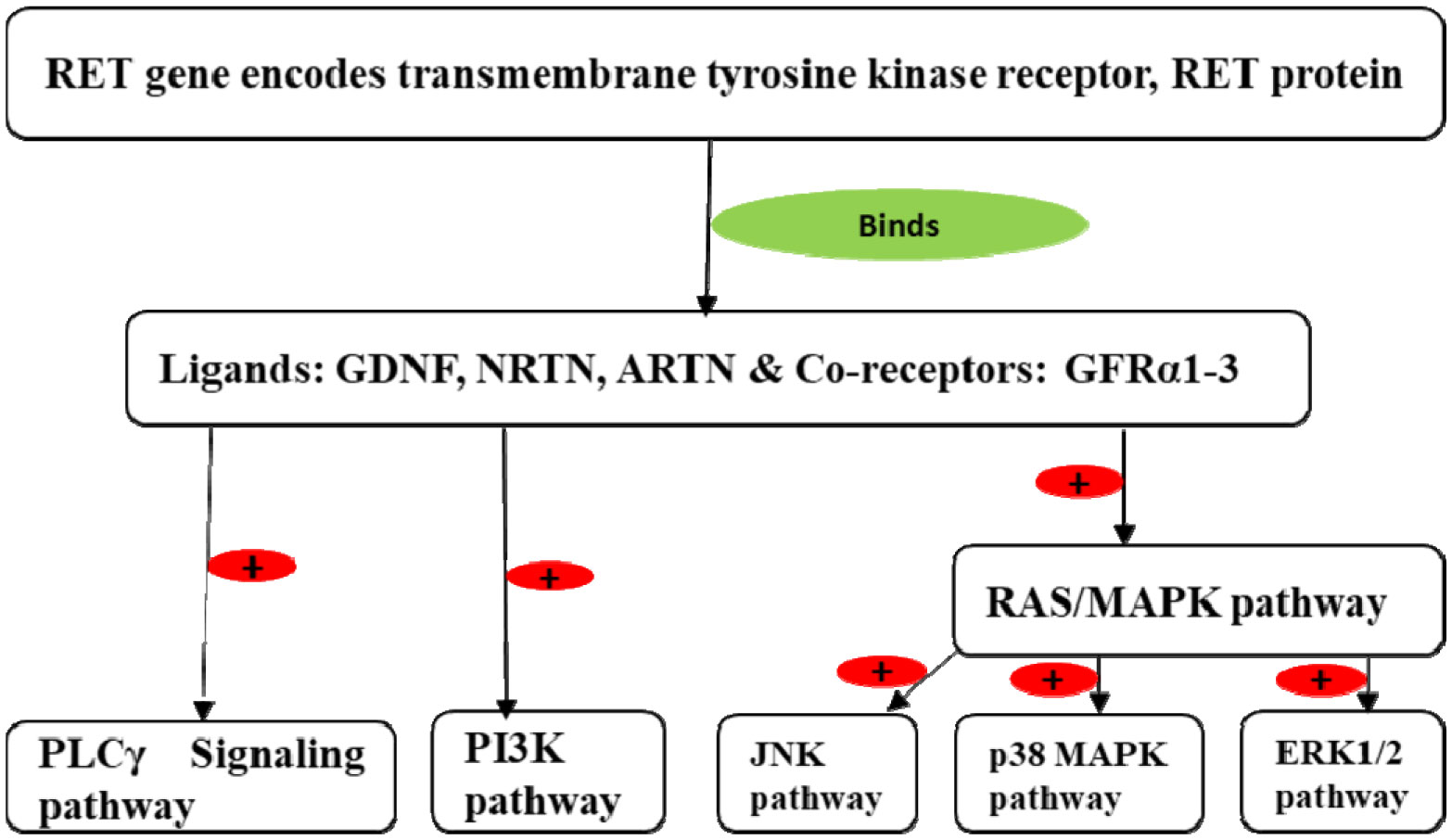
Metal matrix composites (MMCs) have been a fundamental element in the development of technologies related to the aerospace and automotive industries. This is because they have an excellent weight-to-strength ratio, i.e., they are light materials with a high mechanical resistance. In the manufacturing of MMCs, the incorporation and homogeneous dispersion of reinforcements in the matrix has been one of the biggest challenges. The issue has expanded to the manufacturing of materials reinforced with nano-scaled particles. This study is aimed at the manufacturing, optimization, and characterization of the polymeric matrix reinforced with carbon nanotubes and alumina (hybrid composites), in order to use the polymeric matrix as an inclusion vehicle of the nano-reinforcements in a metallic matrix.
The synthesis of the polymeric matrix composites was carried out by a solution mixing technique using polyvinyl alcohol as a matrix. For the reinforcement's dispersion, a magnetic stirring and sonication were used. Finally, the solution was put into a petri dish to allow its polymerization. The nano-reinforcement dispersion qualification and the quantification of the polymer matrix composite were carried out through the tension, nanoindentation, dynamical mechanical analysis test, elastic modulus mapping, and statistical model for dispersion. In addition, a preliminary study of the metallic composite was carried out and was fabricated by the sandwich technique. The initial characterization of the composites was performed through the nanoindentation test.
Citation: Carlos A. Sánchez, Yamile Cardona-Maya, Andrés D. Morales, Juan S. Rudas, Cesar A. Isaza. Development and evaluation of polyvinyl alcohol films reinforced with carbon nanotubes and alumina for manufacturing hybrid metal matrix composites by the sandwich technique[J]. AIMS Materials Science, 2021, 8(2): 149-165. doi: 10.3934/matersci.2021011
[1] | Dai Mitsushima . Contextual Learning Requires Functional Diversity at Excitatory and Inhibitory Synapses onto CA1 Pyramidal Neurons. AIMS Neuroscience, 2015, 2(1): 7-17. doi: 10.3934/Neuroscience.2015.1.7 |
[2] |
Frank O. Bastian .
Is Alzheimers Disease Infectious? Relative to the CJD Bacterial Infection Model of Neurodegeneration. AIMS Neuroscience, 2015, 2(4): 240-258. doi: 10.3934/Neuroscience.2015.4.240 |
[3] | Mary B. Dratman, Joseph V. Martin . The many faces of thyroxine. AIMS Neuroscience, 2020, 7(1): 17-29. doi: 10.3934/Neuroscience.2020002 |
[4] | Vandana Rai, Pradeep Kumar . Methylenetetrahydrofolate reductase (MTHFR) gene C677T (rs1801133) polymorphism and risk of alcohol dependence: a meta-analysis. AIMS Neuroscience, 2021, 8(2): 212-225. doi: 10.3934/Neuroscience.2021011 |
[5] | Khue Vu Nguyen . Encephalomalacia/gliosis, deep venous thrombosis, and cancer in Arg393His antithrombin Hanoi and the potential impact of the β-amyloid precursor protein (APP) on thrombosis and cancer. AIMS Neuroscience, 2022, 9(2): 175-215. doi: 10.3934/Neuroscience.2022010 |
[6] | John Bickle . The first two decades of CREB-memory research: data for philosophy of neuroscience. AIMS Neuroscience, 2021, 8(3): 322-339. doi: 10.3934/Neuroscience.2021017 |
[7] | Khue Vu Nguyen . Potential molecular link between the β-amyloid precursor protein (APP) and hypoxanthine-guanine phosphoribosyltransferase (HGprt) enzyme in Lesch-Nyhan disease and cancer. AIMS Neuroscience, 2021, 8(4): 548-557. doi: 10.3934/Neuroscience.2021030 |
[8] | Chiyoko Kobayashi Frank . Reviving pragmatic theory of theory of mind. AIMS Neuroscience, 2018, 5(2): 116-131. doi: 10.3934/Neuroscience.2018.2.116 |
[9] | Georgia Theocharopoulou . The ubiquitous role of mitochondria in Parkinson and other neurodegenerative diseases. AIMS Neuroscience, 2020, 7(1): 43-65. doi: 10.3934/Neuroscience.2020004 |
[10] | Thomas M. H. Hope . The Bilingual Cognitive Advantage: No Smoke without Fire?. AIMS Neuroscience, 2015, 2(2): 58-65. doi: 10.3934/Neuroscience.2015.2.58 |
Metal matrix composites (MMCs) have been a fundamental element in the development of technologies related to the aerospace and automotive industries. This is because they have an excellent weight-to-strength ratio, i.e., they are light materials with a high mechanical resistance. In the manufacturing of MMCs, the incorporation and homogeneous dispersion of reinforcements in the matrix has been one of the biggest challenges. The issue has expanded to the manufacturing of materials reinforced with nano-scaled particles. This study is aimed at the manufacturing, optimization, and characterization of the polymeric matrix reinforced with carbon nanotubes and alumina (hybrid composites), in order to use the polymeric matrix as an inclusion vehicle of the nano-reinforcements in a metallic matrix.
The synthesis of the polymeric matrix composites was carried out by a solution mixing technique using polyvinyl alcohol as a matrix. For the reinforcement's dispersion, a magnetic stirring and sonication were used. Finally, the solution was put into a petri dish to allow its polymerization. The nano-reinforcement dispersion qualification and the quantification of the polymer matrix composite were carried out through the tension, nanoindentation, dynamical mechanical analysis test, elastic modulus mapping, and statistical model for dispersion. In addition, a preliminary study of the metallic composite was carried out and was fabricated by the sandwich technique. The initial characterization of the composites was performed through the nanoindentation test.
The enteric nervous system is part of the autonomic nervous system and is comprised of a complex array of interconnected neurons in ganglia located throughout the gut wall. The majority of neurons of the gut wall are derived from the vagal neural crest cells, with a minor contribution from the sacral neural crest cells [1]–[3]. These neural crest cells undergo massive migration, proliferation, and differentiation, an event that starts at about embryonic day 8.5 in animals and after the third week of intrauterine life in humans [4]. Several genes play an important role during the development of enteric neurons including the Rearranged During Transfection (RET) gene [5].
The enteric nervous system is derived from the vagal and sacral neural crest of somite levels 1–7 and 28 [6]. These crest cells give rise to enteric neurons and ganglia of the pre-umbilical and post-umbilical parts of the gut wall [7]. They initially undergo a single wave of rostrocaudal migration along the gut wall [8],[9]. Only a small number of neural crest cells are required for rostrocaudal colonization in the gut wall [10],[11]. Next, they migrate to the unoccupied site of the developing gut and proliferate [12]. The migration of immature enteric neuroblasts in the gut wall takes place, on average, at a speed of 15 µm/h [13], which is slow compared to the undifferentiated vagal enteric neural crest cells [13],[14]. A second wave of migration occurs from the periphery to the deep layers of the gut wall through connective tissue pathways, forming the myenteric plexus first, followed by the submucosal plexus [15]. In birds, the enteric neural crest cells first migrate toward the submucosa, forming the submucosal plexus, which then migrates outwards between muscle layers to form the myenteric plexus [16]. During the morphogenesis and differentiation of enteric neural crest cells into enteric neurons, several proteins [17],[18] play essential roles. For the differentiation of vagal and sacral neural crest cells into enteric neurons, the RET gene and the encoded RET protein play a pivotal role [19],[20].
The RET protein is a receptor tyrosine kinase. The RET gene was originally described as a human oncogene, but it was later established that RET plays a crucial role in the development of enteric neurons and defects in the human RET gene result in the syndrome known as Hirschsprung's disease [21].
The RET gene lies in the long arm of chromosome 10 (10q11.2) and contains 21 exons [22] and 18 or 5 introns [23],[24]. The DNA sequence of this gene was originally found to be rearranged within 3T3 fibroblast cell line following transfection with DNA from lymphoma cells [25].
The RET gene encodes a receptor tyrosine kinase transmembrane protein [26]. The RET protein has three different isoforms (RET51, RET43, and RET9), which differ in the C-terminal amino acids [27]. Two isoforms, RET9 and RET51, also differ in their intracellular domains [28],[29]. RET is comprised of 1114 amino acids [30] and has three domains. The N-terminal domain is extracellular and consists of 29–635 amino acids [30]. It has four cadherin-like domains (CLDs) and cysteine-rich regions [31]–[33]. The CLDs each consist of 110 amino acids [34] and CLD2 and CLD3 each have a Ca2+ binding site, which is required for maintaining the integrity of the RET protein [35]. The cysteine-rich regions contain 120 residues and are connected to the transmembrane domain [34]. The hydrophobic transmembrane domain of RET spans the cell membrane [31],[33] and consists of 636–657 amino acids [30]. It mediates extracellular calcium-binding for maturation of the immature 150-kDa RET protein in the endoplasmic reticulum to the mature 170-kDa protein and its migration to the cell membrane [36],[37]. Finally, the cytoplasmic domain is a tyrosine (Tyr) kinase domain and consists of 657–1114 amino acids [30]. It contains 16 tyrosine residues (six in RET9, 18 in RET51, whereas Tyr 1090 and Tyr 1096 are present only in RET51) [31],[32]. This domain also contains catalytic protein kinases, a distinct regulatory sequence of 14–18 tyrosine residues, and serine and threonine phosphorylation sites [38],[39]. There are 18 tyrosine residues, two in the juxtamembrane domain, 11 in the kinase domain, and five in the carboxyl terminal tail [40]. In addition, this domain also has phosphopeptide motifs that provide a binding and docking site for cytoplasmic downstream signaling proteins, such as Src homolog 2 (SH2) and phosphotyrosine-binding domain (PTB) [41].
The RET protein is a member of the glial cell-derived neurotrophic factor (GDNF) family of extracellular signaling molecules [25],[42]. The RET ligand GDNF [1],[43] is a dimeric growth factor protein related to a member of the transforming growth factor-beta (TGF-β) superfamily. This superfamily has four additional subtypes: GDNF, neurturin (NRTN) [44], persephin (PSPN) [45], and artemin (ARTN) [46]. They bind with the RET protein via its co-receptors, the GDNF receptor alpha proteins (glycosylphosphatidylinositol (GPI) anchored co-receptor family) GFRα1, GFRα2, GFRα3, and GFRα4 [47]–[49]. The cysteine-rich extracellular CLD4 domain of RET makes a direct crosslink with GFRα1 [50] and the CLD1–3 domains [51] fold into a compact shell [52]. This maintains the conformation of RET during binding [50]. The extracellular domains form ternary complexes of their ligand, co-receptor, and the receptor RET protein as follows: i) GDNF with GFRα1 and RET; ii) NRTN with GFRα2 and RET; iii) ARTN with GFRα3 and RET. These ternary complexes induce dimerization of the RET protein. During dimerization, there is trans-autophosphorylation of Tyr905 and Tyr900 of the tyrosine kinase domain of the RET protein, which further autophosphorylates other tyrosine residues (Tyr981, Tyr1015, Tyr1062, Tyr1063, and Tyr1096) [39],[53]. Phosphorylation of Tyr1096 takes place only in the RET51 isoform. Phosphorylation of Tyr1062 of the tyrosine kinase domain of the RET protein activates RAS/MAPK and PI3K-PKB/AKT pathways [40],[41],[54],[55] while autophosphorylation of other tyrosine residues induces PLC-γ and JNK pathways [47] (Figure 1).
Phosphorylation of serine, threonine, and tyrosine of the activation loop of the tyrosine kinase domain of the RET protein stimulates mitogen-activated protein kinase kinases (MAPKK), which are upstream of the MEK proteins. MEKs are activated by various upstream activators, including kinases and small GTP binding proteins. MEK then activates three MAPK [56] pathways: extracellular signal-regulated kinase 1/2 (ERK1/2), JNK, and p38 mitogen-activated protein kinase (p38 MAPK) (Figure 2). All three pathways consist of three-tiered kinase cascades that phosphorylate hundreds of substrates in the cytoplasm and nucleus, leading to cellular proliferation, survival, apoptosis, migration, and differentiation [57].
Upon activation, the RET protein tyrosine kinase domain [58] binds to Src homolog 2 and 3 (SH2 and SH3) domains of phosphotyrosine. SH2 and SH3 bind to adaptor molecule GRB2 [59],[60], which interacts with the guanine nucleotide exchange factor (GEF) Sos (Son of sevenless) [61]. Sos then promotes the exchange of GDP for GTP on the RAS protein, which binds and activates the MAPKK kinase protein, RAF. RAF then phosphorylates threonine and tyrosine residues on the activation loop of the MAPK protein, ERK1/2, which further phosphorylates multiple cytoplasmic and cytoskeletal proteins [56],[62] such as MAPK-activated protein kinases and ribosomal S6 kinases (RSK). ERK and ribosomal S6 kinases 1/2 (RSK1/2) then translocate into the nucleus. ERK phosphorylates and activates several transcription factors, including SP, E2F, ELK-1, AP-1 [63], ELK-7, FOS, Myc, and MEF2 [62],[64],[65]. RSK1/2 activates big MAP kinase (BMK1), i.e., ERK5 [62],[66], and it phosphorylates several transcription factors including Myc, MEF2 family members, FOS, and serum- and glucocorticoid-inducible kinase (SGK). Together, these transcription factors lead to cell cycle progression [67], proliferation, survival, migration, and fate choice of cells [68],[69].
The JNK pathway is required for the normal migration of enteric neural crest cells. Several MAPKKKs together with MEKK1–4, MLK3, and Tak1 phosphorylate and activate MKK4 and MKK7 [70]. MKK4 and MKK7 then catalyze the phosphorylation of C-Jun N-terminal kinase (CJN Kinase) [71]. This further activates MAPKKK via the small G-protein, RAC. RAC further activates MLK3, MEKK1, and MEKK4 [72]–[74], and finally activates the JNK pathway. This JNK pathway then causes the phosphorylation and activation of several transcription factors, including C-Jun, Jun A, Jun B, ATF2, and EIK, and these enable enteric neural crest cell survival [70] and migration [75],[76].
Upon phosphorylation of the tyrosine kinase domain of the RET protein, it activates the four p38 isoforms, α, β, γ, and δ [77]. These p38 isoforms activate several MAPKKKs, including MEK1–4 and MLK1–4, which further activate MKK3 and MKK6, and thus induce apoptosis of extraneural crest cells [71].
PLCγ contains two SH2 domains and one SH3 domain. The SH2 domains bind phosphotyrosine and the SH3 domain binds the proline-rich sequences of RET [78],[79]. Upon ligand (GDNF) stimulation, there is phosphorylation of Tyr1015 and Tyr1016 of the tyrosine kinase domain of the RET protein. Through the PLCγ binding domain [80], the RET protein recruits the transmembrane adaptor, CAT protein [81]. CAT activates calcium calmodulin-dependent kinase II (CAMK II) and ERK1/2 [82],[83], which causes the release of Ca2+ from the endoplasmic reticulum and extracellular milieu [84],[85] through the inositol 1,4,5-triphosphate (InsP3) receptor (InsPR). The released Ca2+ then triggers RAS/MAPK by phosphorylating p42/44 of MAPK (ERK1/2). This modulates the enteric neuronal migration and enteric neuron synaptic plasticity [85],[86] (Figure 3. a).
Upon stimulation by its ligand (GDNF), the tyrosine kinase domain of the RET protein binds with regulatory subunits p85α, p55α, p50α, p85β, p55γ, p110α, and p110β of PI3K enzymes via its phosphotyrosine-binding SH2 domain [87]. Then, the catalytic subunits p110α (activated by G-protein RAS) and p110β (activated by G-protein RAC) [88] degrade the phosphatidylinositol (3,4,5)-trisphosphate (PIP3) to phosphatidylinositol (4,5)-bisphosphate (PIP2) by phosphatase [89]. This PIP2 activates 3-phosphoinositide-dependent protein kinase-1 (PDPK1/PDK1) [90] and phosphorylates AKT [91],[92]. The activated AKT then regulates neural crest cell survival, specification, migration, proliferation, and differentiation into enteric neuroblasts [93] via the mTorc and P53 pathways [94] (Figure 3. b).
Deletion of the RET gene or mutations in the exons and introns that result in changes in the intracellular and extracellular domains of RET lead to Hirschsprung's disease (Tables 1–11).
Mutations | Domains affected | Exons affected | Introns affected | Codons affected | Outcomes |
Germline mutation [95],[96] | Extracellular domain [95],[96] | 10 | - | 609, 611, 618, 620 | Hirschsprung's disease |
11 | - | 630, 634 | |||
Intracellular domain-tyrosine kinase 1 residue of tyrosine kinase domain [95],[96] | 15 | - | 883, 891 | ||
14 | - | 804 | |||
15 | - | 883, 891, 918 | |||
Germline mutation [97] | - | 10 | - | c.1852 T>C |
Mutations | Domains Affected | Exons affected | Introns affected | Codons affected | Outcomes |
Nonsense mutation | Extracellular domain [98] | 2, 3, 4, 5, 6 [98] | - | - | Hirschsprung's disease |
Amino acid substitution [98]–[101] | - | - | - | Familial or sporadic cases of Hirschsprung's disease | |
Frameshift mutation | RET gene | - | - | Phe147del [102] | Hirschsprung's disease |
Amino acid substitution in protein truncation of RET [98]–[101] | - | - | - | Familial or sporadic cases of Hirschsprung's disease | |
Point mutation | RET gene in heterozygous state [24],[98],[103] | - | - | - | Hirschsprung's disease |
Mutations | Domains Affected | Exons affected | Introns affected | Codons affected | Outcomes |
Missense mutation | Extracellular domain [98] | 2, 3, 4, 5, 6 [98] | - | - | Hirschsprung's disease |
Impair the RET kinase activity leading to the impairment of the phospholipase C-γ signaling pathway [104] | - | - | E762Q, S767R, R972G, M900T [104] | ||
Complete loss of RET kinase activity [104] | - | - | S765P, R873Q, F893L, R897Q, E921K [104] | ||
RET tyrosine kinase domain [23] | - | - | - | ||
Dominant negative effect through loss of function [24],[105],[106] | - | - | - | ||
- | 15 [107] | - | At nucleotide 2813G to A with R873Q exchange in codon 873 [107] | ||
3 [108] | - | Nucleotide change GTG to ATG (V202M mutation) [108] | |||
7 [108] | - | Nucleotide change GAA to AAA (E480K mutation) [108] | Rectosigmoidal aganglionosis | ||
17 [108] | - | Nucleotide change CCA to ATA (P973L mutation) [108] | |||
13 [108] | - | Nucleotide change GAC to AAC (D77/N mutation) [108] | Total gut wall aganglionosis | ||
Amino acid substitution in RET protein [98]–[101] | - | - | - | Familial or sporadic cases of Hirschsprung's disease |
Mutations | Locations | Outcomes |
Deletion [103] | RET gene [103] | Hirschsprung's disease In 20% patient have low efficiency in detection of deletion [103] |
Partial deletion [109] | RET locus at pericentromeric region of chromosome 10 [109] | |
Interstitial deletion [110] | In the long arm of chromosome 10- del10(q11.21, q21.2) [110] | Total colonic aganglionosis and minor involvement of myenteric plexus [110] |
Proximal deletion [111] | In the long arm 10- del10q11.2 to q21.2 Deletion location likely lying between loci D10S208 and D10S196 [111] |
Colonic aganglionosis in hindgut [111]. |
Cytogenetic deletion [112] | del (10) (q11.2 to q21.2) [112] | Total aganglionosis with small bowel involvement [112] |
Mutations | Locations | Effects | Outcomes |
Mutation in extracellular domain | N terminus region of RET protein [113]–[115] | Affect the amino acid residue No glycosylation of immature 150-kDa form in the endoplasmic reticulum No production of mature 170k-Da form of RET protein No expression of RET protein in cell membrane [113],[114] |
Hirschsprung's disease [113]–[115] |
Mutations | Locations | Effects | Outcomes |
Mutation in intracellular domain | Tyrosine kinase domain [23] | Impaired intracellular signaling pathways [23] | Hirschsprung's disease |
Tyrosine kinase domain 1 [Glu 762-Gln (E762Q), Ser65 to Pro (S765P) and Ser767 to Arg (S767R)] or tyrosine kinase domain 2 [Arg 873 to Gln (R897Q), Glu 921 to Lys (E921K), Arg 972 to Gly (R972G), Pro 973 to Leu (P973L) and Met 980 to Thr (M980T)] [24],[98]–[100],[116]–[119] | - | Familial and sporadic Hirschsprung's disease [24],[98]–[100],[116]–[119] | |
Tyrosine residue at position 1062 which is intracytoplasmic docking site of RET protein [120] | Impaired fixation of SHc to RET protein and thus prevention of the phosphorylation and inhibition of the signaling pathway and thus exert negative effect in the enteric neurogenesis [120] | Hirschsprung's disease |
Expression of RET gene | Effects | Outcomes |
Insufficient level of expression | Insufficient expression of RET protein on the cell surface for GDNF and its co-receptor GFR α1–4 [101],[121] | Hirschsprung's disease |
Mutations | Locations | Effects | Outcomes |
Mutation in exons | Exon 2 in codon 32 changing CTG to TTG [98] | Changes the protein sequence of extracellular domain of RET from serine to leucine [98] | Congenital absence of enteric neurons and ganglia in intestine [98] |
Exon 3 in codon 180 changing CGA to TGA [98] | Changes the protein sequence of extracellular domain of RET from arginine to stop codon [98]. | ||
Exon 5 in codon 330 changing CGG to CAG [98] | Changes the protein sequence in extracellular domain of RET from arginine to glutamine [98] | ||
Exon 6 in codon 393 changing TTC to TTA [98] | Changes the protein sequence of extracellular domain of RET from phenylalanine to leucine [98] | ||
Exon 10 with nucleotide change of C1876A and amino acid change of Q6226K [122] | - | Sporadic ultra-short-segment aganglionosis [122] | |
Exon 11 with nucleotide change of C1941T and amino acid change of 16471 [122] | - | Sporadic long-segment aganglionosis [122] | |
Exon 10 with change in five cysteine codons from Cys to Trp at codon 699 and Cys to Arg at codon 618 or 620 [97] | - | Hirschsprung's disease | |
Exon 2 with change in nucleotide from C254 G to A [123] | Loss of function of RET gene [123] | Total colonic aganglionosis [123] | |
Exon 13 with change in nucleotide from C2308 C to T [123] | |||
Exon 14 with change in nucleotide from C2578 C to T [123] | |||
Exon 4 with change in nucleotide from C789 C to G [123] | - | Long segment Hirschsprung's [123] |
Mutations | Effects | Outcomes |
RET isoforms mutations and defects | RET9 [124] | Lack of enteric ganglion in colon [124] |
Mutation of tyrosine 1062 of RET9 to phenylalanine [125] | Deficient in enteric nervous system [125] |
Mutations | Locations | Effects | Outcomes |
Mutation of enhancer [126] | Enhancer domain of the RET gene in intron 1 (CrS2435357) [126] | - | Hirschsprung's disease with significantly higher impact in males than females [126] |
Promoter defect [127] | Methylation of promoter of RET has 5′ CC-3′ [127] | - | Colonic aganglionosis [127] |
Mutation in introns | Alteration in intron 4 at putative branch site of 24 nucleotides in front of exon 15 with nucleotide exchange of G to A [107] | - | Hirschsprung's disease [107] |
Missense mutation in intron 19 (IVS 19-19 C/T) [108] | - | Only rectosigmoidal aganglionosis [108] |
Mutations | Locations | Effects | Outcomes |
Homozygous RET mutations | C620R mutation [128] | - | Hirschsprung's diseases [128] |
Mutation of tyrosine 1062 in RET with phenylalanine [129] | Impairing the binding site of tyrosine 1062 for phosphotyrosine-binding domains for several adaptors and effector proteins which otherwise are important for activation of intracellular signaling pathways, such as RAS/ERK, phosphatidylinositol 3-kinase/AKT, and Jun-associated N-terminal kinase pathways [129] | Severe defect in the development of enteric nervous system in 40% of cases [129] | |
Homozygous missense mutation (CGG to TGG) at codon 969 of RET with amino acid change from arginine to tryptophan [130] | Critical alteration in RET tyrosine kinase activity [130] | Total gastrointestinal tract aganglionosis [130] | |
Heterozygous RET mutation [128] | C620R mutation [128] | - | Hirschsprung's disease including hypoganglionosis of gastrointestinal tract [128] |
Vagal and sacral neural crest cells migrate in a rostrocaudal direction where they colonize in an orderly manner in the foregut, midgut, and hindgut following signaling by the receptor tyrosine kinase RET protein. This protein promotes the survival of enteric neurons, as well as proliferation and differentiation of multipotent enteric progenitor cells present in the gut wall. Developmental studies in model organisms and genetic studies of Hirschsprung's disease have provided a detailed understanding of enteric nervous system development via expression of the RET gene. In summary, the RET gene encodes a tyrosine kinase receptor, RET, which is required for the normal formation of enteric neurons. Mutation of the RET gene leads to dysfunctional RET binding to the GDNF, ARTN, and NRTN ligands resulting in Hirschsprung's disease.
[1] |
Isaza MCA, Ledezma Sillas JE, Meza JM, et al. (2017) Mechanical properties and interfacial phenomena in aluminum reinforced with carbon nanotubes manufactured by the sandwich technique. J Compos Mater 51: 1619-1629. doi: 10.1177/0021998316658784
![]() |
[2] |
Friedrich K, Almajid AA (2013) Manufacturing aspects of advanced polymer composites for automotive applications. Appl Compos Mater 20: 107-128. doi: 10.1007/s10443-012-9258-7
![]() |
[3] |
Kessler MR (2012) Polymer matrix composites: A perspective for a special issue of polymer reviews. Polym Rev 52: 229-233. doi: 10.1080/15583724.2012.708004
![]() |
[4] |
Isaza C, Sierra G, Meza J (2016) A novel technique for production of metal matrix composites reinforced with carbon nanotubes. J Manuf Sci E-T ASME 138: 024501. doi: 10.1115/1.4030377
![]() |
[5] | Alsharef JM, Taha MR, Khan TA (2017) Physical dispersion of nanocarbons in composites-A review. Jurnal Teknologi 79. |
[6] |
Xin F, Li L (2011) Decoration of carbon nanotubes with silver nanoparticles for advanced CNT/polymer nanocomposites. Compos Part A-Appl S 42: 961-967. doi: 10.1016/j.compositesa.2011.03.024
![]() |
[7] |
LaNasa JA, Torres VM, Hickey RJ (2020) In situ polymerization and polymer grafting to stabilize polymer-functionalized nanoparticles in polymer matrices. J Appl Phys 127: 134701. doi: 10.1063/1.5144212
![]() |
[8] |
Khodabakhshi F, Simchi A (2017) The role of microstructural features on the electrical resistivity and mechanical properties of powder metallurgy Al-SiC-Al2O3 nanocomposites. Mater Design 130: 26-36. doi: 10.1016/j.matdes.2017.05.047
![]() |
[9] |
Khandelwal A, Mani K, Srivastava N, et al. (2017) Mechanical behavior of AZ31/Al2O3 magnesium alloy nanocomposites prepared using ultrasound assisted stir casting. Compos Part B-Eng 123: 64-73. doi: 10.1016/j.compositesb.2017.05.007
![]() |
[10] |
Kawasaki M, Han JK, Lee DH, et al. (2018) Fabrication of nanocomposites through diffusion bonding under high-pressure torsion. J Mate Res 33: 2700-2710. doi: 10.1557/jmr.2018.205
![]() |
[11] |
Li Q, Yuan X, Xu H, et al. (2019) Microstructure and fracture toughness of in-situ nanocomposite coating by thermal spraying of Ti3AlC2/Cu powder. Ceram Int 45: 13119-13126. doi: 10.1016/j.ceramint.2019.03.246
![]() |
[12] |
Merino CAI, Sillas JL, Meza J, et al. (2017) Metal matrix composites reinforced with carbon nanotubes by an alternative technique. J Alloys Compd 707: 257-263. doi: 10.1016/j.jallcom.2016.11.348
![]() |
[13] |
Isaza MCA, Herrera RamƖrez J, Ledezma Sillas J, et al. (2018) Dispersion and alignment quantification of carbon nanotubes in a polyvinyl alcohol matrix. J Compos Mater 52: 1617-1626. doi: 10.1177/0021998317731151
![]() |
[14] |
Yuan W, Cui J, Xu S (2016) Mechanical properties and interfacial interaction of modified calcium sulfate whisker/poly(vinyl chloride) composites. J Mater Sci Technol 32: 1352-1360. doi: 10.1016/j.jmst.2016.05.016
![]() |
[15] | Srivatsan T, Lin Y, Chen F, et al. (2018) Synthesis and microstructural development of particulate reinforced metal-matrix composites using the technique of spray atomization and deposition, TMS Annual Meeting & Exhibition, Springer, Cham, 149-182. |
[16] |
Koli DK, Agnihotri G, Purohit R (2014) A review on properties, behaviour and processing methods for Al-nano Al2O3 composites. Procedia Mater Sci 6: 567-589. doi: 10.1016/j.mspro.2014.07.072
![]() |
[17] |
Luo H, Dong J, Xu X, et al. (2018) Exploring excellent dispersion of graphene nanosheets in three-dimensional bacterial cellulose for ultra-strong nanocomposite hydrogels. Compos Part A-Appl S 109: 290-297. doi: 10.1016/j.compositesa.2018.03.007
![]() |
[18] |
Salom C, Prolongo M, Toribio A, et al. (2018) Mechanical properties and adhesive behavior of epoxy-graphene nanocomposites. Int J Adhes Adhes 84: 119-125. doi: 10.1016/j.ijadhadh.2017.12.004
![]() |
[19] |
Hu X, Ren N, Chao Y, et al. (2017) Highly aligned graphene oxide/poly(vinyl alcohol) nanocomposite fibers with high-strength, antiultraviolet and antibacterial properties. Compos Part A-Appl S 102: 297-304. doi: 10.1016/j.compositesa.2017.08.015
![]() |
[20] | Karthikeyan P, Babu BG, Sabarinathan C, et al. (2015) Tribological performance of carbon nanotubes-alumina hybrid/epoxy composites. Optoelectron Adv Mater-Rapid Commun 9: 455-459. |
[21] | Karthikeyan P, Babu B, Siva K, et al. (2016) Experimental investigation on mechanical behavior of carbon nanotubes-Alumina hybrid epoxy nanocomposites. Dig J Nanomater Bios 11: 625-632. |
[22] |
Dabees S, Kamel BM, Tirth V, et al. (2020) Experimental design of Al2O3/MWCNT/HDPE hybrid nanocomposites for hip joint replacement. Bioengineered 11: 679-692. doi: 10.1080/21655979.2020.1775943
![]() |
[23] |
Jiang D, Zhang J, Lv Z (2012) Multi-wall carbon nanotubes (MWCNTs)-SiC composites by laminated technology. J Eur Ceram Soc 32: 1419-1425. doi: 10.1016/j.jeurceramsoc.2011.07.035
![]() |
[24] |
Ahmad I, Ahmed S, Subhani T, et al. (2016) Synergic influence of MWCNTs and SiC nanoparticles on the microstructure and properties of Al2O3 ceramic hybrid nanocomposites. Curr Appl Phys 16: 1649-1658. doi: 10.1016/j.cap.2016.10.009
![]() |
[25] |
Han D, Mei H, Xiao S, et al. (2018) A review on the processing technologies of carbon nanotube/silicon carbide composites. J Eur Ceram Soc 38: 3695-3708. doi: 10.1016/j.jeurceramsoc.2018.04.033
![]() |
[26] |
Padmavathi K, Ramakrishnan R (2014) Tribological behaviour of aluminium hybrid metal matrix composite. Procedia Eng 97: 660-667. doi: 10.1016/j.proeng.2014.12.295
![]() |
[27] |
Kim H, Babu J, Kang C (2013) Fabrication of A356 aluminum alloy matrix composite with CNTs/Al2O3 hybrid reinforcements. Mat Sci Eng A-Struct 573: 92-99. doi: 10.1016/j.msea.2013.02.041
![]() |
[28] | Paramsothy M, Chan J, Kwok R, et al. (2012) TiC nanoparticle addition to enhance the mechanical response of hybrid magnesium alloy. J Nanotechnol 2012: 401574. |
[29] |
Wei TZ, Shamsuri SRB, Yee CS, et al. (2013) Effect of sliding velocity on wear behavior of magnesium composite reinforced with SiC and MWCNT. Procedia Eng 68: 703-709. doi: 10.1016/j.proeng.2013.12.242
![]() |
[30] | Zhou X, Su D, Wu C, et al. (2012) Tensile mechanical properties and strengthening mechanism of hybrid carbon nanotube and silicon carbide nanoparticle-reinforced magnesium alloy composites. J Nanomater 2012: 851862. |
[31] | Ramirez JMH, Bustamante RP, Merino CAI, et al. (2020) Unconventional Techniques for the Production of Light Alloys and Composites, Springer, Cham. |
[32] | SIGMA-ALDRICH Products: Poly(vinyl alcohol) (363081), 2021. Available from: https://www.sigmaaldrich.com/. |
[33] |
Rossell MD, Kuebel C, Ilari G, et al. (2013) Impact of sonication pretreatment on carbon nanotubes: A transmission electron microscopy study. Carbon 61: 404-411. doi: 10.1016/j.carbon.2013.05.024
![]() |
[34] |
Chen J, Bull S (2006) On the relationship between plastic zone radius and maximum depth during nanoindentation. Surf Coat Tech 201: 4289-4293. doi: 10.1016/j.surfcoat.2006.08.099
![]() |
[35] |
Luo Z, Koo JH (2007) Quantifying the dispersion of mixture microstructures. J Microsc 225: 118-125. doi: 10.1111/j.1365-2818.2007.01722.x
![]() |
[36] |
Olayo R, GarcƖa E, GarcƖa-Corichi B, et al. (1998) Poly(vinyl alcohol) as a stabilizer in the suspension polymerization of styrene: The effect of the molecular weight. J Appl Polym Sci 67: 71-77. doi: 10.1002/(SICI)1097-4628(19980103)67:1<71::AID-APP8>3.0.CO;2-L
![]() |
[37] |
More S, Dhokne R, Moharil S (2018) Structural properties and temperature dependence dielectric properties of PVA-Al2O3 composite thin films. Polym Bull 75: 909-923. doi: 10.1007/s00289-017-2069-0
![]() |
[38] |
Mallakpour S, Dinari M (2013) Nanocomposites of poly(vinyl alcohol) reinforced with chemically modified Al2O3: Synthesis and characterization. J Macromol Sci B 52: 1651-1661. doi: 10.1080/00222348.2013.789349
![]() |
[39] |
Fan B, He D, Liu Y, et al. (2017) Influence of thermal treatments on the evolution of conductive paths in carbon nanotube-Al2O3 hybrid reinforced epoxy composites. Langmuir 33: 9680-9686. doi: 10.1021/acs.langmuir.6b03915
![]() |
[40] |
Li W, Dichiara A, Zha J, et al. (2014) On improvement of mechanical and thermo-mechanical properties of glass fabric/epoxy composites by incorporating CNT-Al2O3 hybrids. Compos Sci Technol 103: 36-43. doi: 10.1016/j.compscitech.2014.08.016
![]() |
[41] |
Li W, He D, Dang Z, et al. (2014) In situ damage sensing in the glass fabric reinforced epoxy composites containing CNT-Al2O3 hybrids. Compos Science Technol 99: 8-14. doi: 10.1016/j.compscitech.2014.05.005
![]() |
[42] |
Haslam MD, Raeymaekers B (2013) A composite index to quantify dispersion of carbon nanotubes in polymer-based composite materials. Composites Part B-Eng 55: 16-21. doi: 10.1016/j.compositesb.2013.05.038
![]() |
[43] |
Luo Z, Koo J (2008) Quantitative study of the dispersion degree in carbon nanofiber/polymer and carbon nanotube/polymer nanocomposites. Mater Lett 62: 3493-3496. doi: 10.1016/j.matlet.2008.03.010
![]() |
1. | Tomasz Kucharczyk, Paweł Krawczyk, Dariusz M. Kowalski, Adam Płużański, Tomasz Kubiatowski, Ewa Kalinka, RET Proto-Oncogene—Not Such an Obvious Starting Point in Cancer Therapy, 2022, 14, 2072-6694, 5298, 10.3390/cancers14215298 | |
2. | Jianhua Mu, Yuxi Zhang, Guoying Liao, Xinxin Li, Yinyan Luo, Zhaorong Huang, Caiyun Luo, Kai Wu, Association of rs2435357 and rs2506030 polymorphisms in RET with susceptibility to hirschsprung disease: A systematic review and meta-analysis, 2022, 10, 2296-2360, 10.3389/fped.2022.1030933 | |
3. | Chacchu Bhattarai, Phanindra P. Poudel, Arnab Ghosh, Sneha G. Kalthur, Comparative role of SOX10 gene in the gliogenesis of central, peripheral, and enteric nervous systems, 2022, 128, 03014681, 13, 10.1016/j.diff.2022.09.001 | |
4. | Claudia Ricci, Antonia Salvemini, Cristina Dalmiglio, Maria Grazia Castagna, Silvia Cantara, From Circulating Tumor Cells to Mirna: New Challenges in the Diagnosis and Prognosis of Medullary Thyroid Cancer, 2023, 15, 2072-6694, 4009, 10.3390/cancers15154009 | |
5. | Katarzyna Stawarz, Monika Durzynska, Adam Galazka, Monika Paszkowska, Karolina Bienkowska-Pluta, Jakub Zwolinski, Andrzej Tysarowski, Ewa Kwiatkowska, Agnieszka Podgorska, Two sisters diagnosed with familial paraganglioma syndrome type 1 (FPGL1) and multiple endocrine neoplasia type 2A (MEN2A), 2024, 22, 1477-7819, 10.1186/s12957-024-03418-1 | |
6. | N. M. Stepanova, V. A. Novozilov, Yu. I. Petrova, Yu. A. Telnova, Hirschsprung’s disease in newborns: diseases-”Masks”, 2024, 0514-2415, 94, 10.52888/0514-2515-2024-361-2-94-100 | |
7. | Yiran Han, Tiantian Wen, Jia Wang, Jinmiao Shi, Yongqiang Zhu, Preclinical Pharmacokinetics and in vitro Metabolism of FHND5071, a Novel Selective RET Kinase Inhibitor, 2023, 48, 0378-7966, 595, 10.1007/s13318-023-00844-6 | |
8. | M. Namini, G. Bhagya, Manjari Sharma, Personalized Approaches to Lung Cancer Treatment: A Review of Targeted Therapies, Pharmacogenomics, and Combination Strategies, 2025, 29496888, 100073, 10.1016/j.amolm.2025.100073 |
Mutations | Domains affected | Exons affected | Introns affected | Codons affected | Outcomes |
Germline mutation [95],[96] | Extracellular domain [95],[96] | 10 | - | 609, 611, 618, 620 | Hirschsprung's disease |
11 | - | 630, 634 | |||
Intracellular domain-tyrosine kinase 1 residue of tyrosine kinase domain [95],[96] | 15 | - | 883, 891 | ||
14 | - | 804 | |||
15 | - | 883, 891, 918 | |||
Germline mutation [97] | - | 10 | - | c.1852 T>C |
Mutations | Domains Affected | Exons affected | Introns affected | Codons affected | Outcomes |
Nonsense mutation | Extracellular domain [98] | 2, 3, 4, 5, 6 [98] | - | - | Hirschsprung's disease |
Amino acid substitution [98]–[101] | - | - | - | Familial or sporadic cases of Hirschsprung's disease | |
Frameshift mutation | RET gene | - | - | Phe147del [102] | Hirschsprung's disease |
Amino acid substitution in protein truncation of RET [98]–[101] | - | - | - | Familial or sporadic cases of Hirschsprung's disease | |
Point mutation | RET gene in heterozygous state [24],[98],[103] | - | - | - | Hirschsprung's disease |
Mutations | Domains Affected | Exons affected | Introns affected | Codons affected | Outcomes |
Missense mutation | Extracellular domain [98] | 2, 3, 4, 5, 6 [98] | - | - | Hirschsprung's disease |
Impair the RET kinase activity leading to the impairment of the phospholipase C-γ signaling pathway [104] | - | - | E762Q, S767R, R972G, M900T [104] | ||
Complete loss of RET kinase activity [104] | - | - | S765P, R873Q, F893L, R897Q, E921K [104] | ||
RET tyrosine kinase domain [23] | - | - | - | ||
Dominant negative effect through loss of function [24],[105],[106] | - | - | - | ||
- | 15 [107] | - | At nucleotide 2813G to A with R873Q exchange in codon 873 [107] | ||
3 [108] | - | Nucleotide change GTG to ATG (V202M mutation) [108] | |||
7 [108] | - | Nucleotide change GAA to AAA (E480K mutation) [108] | Rectosigmoidal aganglionosis | ||
17 [108] | - | Nucleotide change CCA to ATA (P973L mutation) [108] | |||
13 [108] | - | Nucleotide change GAC to AAC (D77/N mutation) [108] | Total gut wall aganglionosis | ||
Amino acid substitution in RET protein [98]–[101] | - | - | - | Familial or sporadic cases of Hirschsprung's disease |
Mutations | Locations | Outcomes |
Deletion [103] | RET gene [103] | Hirschsprung's disease In 20% patient have low efficiency in detection of deletion [103] |
Partial deletion [109] | RET locus at pericentromeric region of chromosome 10 [109] | |
Interstitial deletion [110] | In the long arm of chromosome 10- del10(q11.21, q21.2) [110] | Total colonic aganglionosis and minor involvement of myenteric plexus [110] |
Proximal deletion [111] | In the long arm 10- del10q11.2 to q21.2 Deletion location likely lying between loci D10S208 and D10S196 [111] |
Colonic aganglionosis in hindgut [111]. |
Cytogenetic deletion [112] | del (10) (q11.2 to q21.2) [112] | Total aganglionosis with small bowel involvement [112] |
Mutations | Locations | Effects | Outcomes |
Mutation in extracellular domain | N terminus region of RET protein [113]–[115] | Affect the amino acid residue No glycosylation of immature 150-kDa form in the endoplasmic reticulum No production of mature 170k-Da form of RET protein No expression of RET protein in cell membrane [113],[114] |
Hirschsprung's disease [113]–[115] |
Mutations | Locations | Effects | Outcomes |
Mutation in intracellular domain | Tyrosine kinase domain [23] | Impaired intracellular signaling pathways [23] | Hirschsprung's disease |
Tyrosine kinase domain 1 [Glu 762-Gln (E762Q), Ser65 to Pro (S765P) and Ser767 to Arg (S767R)] or tyrosine kinase domain 2 [Arg 873 to Gln (R897Q), Glu 921 to Lys (E921K), Arg 972 to Gly (R972G), Pro 973 to Leu (P973L) and Met 980 to Thr (M980T)] [24],[98]–[100],[116]–[119] | - | Familial and sporadic Hirschsprung's disease [24],[98]–[100],[116]–[119] | |
Tyrosine residue at position 1062 which is intracytoplasmic docking site of RET protein [120] | Impaired fixation of SHc to RET protein and thus prevention of the phosphorylation and inhibition of the signaling pathway and thus exert negative effect in the enteric neurogenesis [120] | Hirschsprung's disease |
Mutations | Locations | Effects | Outcomes |
Mutation in exons | Exon 2 in codon 32 changing CTG to TTG [98] | Changes the protein sequence of extracellular domain of RET from serine to leucine [98] | Congenital absence of enteric neurons and ganglia in intestine [98] |
Exon 3 in codon 180 changing CGA to TGA [98] | Changes the protein sequence of extracellular domain of RET from arginine to stop codon [98]. | ||
Exon 5 in codon 330 changing CGG to CAG [98] | Changes the protein sequence in extracellular domain of RET from arginine to glutamine [98] | ||
Exon 6 in codon 393 changing TTC to TTA [98] | Changes the protein sequence of extracellular domain of RET from phenylalanine to leucine [98] | ||
Exon 10 with nucleotide change of C1876A and amino acid change of Q6226K [122] | - | Sporadic ultra-short-segment aganglionosis [122] | |
Exon 11 with nucleotide change of C1941T and amino acid change of 16471 [122] | - | Sporadic long-segment aganglionosis [122] | |
Exon 10 with change in five cysteine codons from Cys to Trp at codon 699 and Cys to Arg at codon 618 or 620 [97] | - | Hirschsprung's disease | |
Exon 2 with change in nucleotide from C254 G to A [123] | Loss of function of RET gene [123] | Total colonic aganglionosis [123] | |
Exon 13 with change in nucleotide from C2308 C to T [123] | |||
Exon 14 with change in nucleotide from C2578 C to T [123] | |||
Exon 4 with change in nucleotide from C789 C to G [123] | - | Long segment Hirschsprung's [123] |
Mutations | Locations | Effects | Outcomes |
Mutation of enhancer [126] | Enhancer domain of the RET gene in intron 1 (CrS2435357) [126] | - | Hirschsprung's disease with significantly higher impact in males than females [126] |
Promoter defect [127] | Methylation of promoter of RET has 5′ CC-3′ [127] | - | Colonic aganglionosis [127] |
Mutation in introns | Alteration in intron 4 at putative branch site of 24 nucleotides in front of exon 15 with nucleotide exchange of G to A [107] | - | Hirschsprung's disease [107] |
Missense mutation in intron 19 (IVS 19-19 C/T) [108] | - | Only rectosigmoidal aganglionosis [108] |
Mutations | Locations | Effects | Outcomes |
Homozygous RET mutations | C620R mutation [128] | - | Hirschsprung's diseases [128] |
Mutation of tyrosine 1062 in RET with phenylalanine [129] | Impairing the binding site of tyrosine 1062 for phosphotyrosine-binding domains for several adaptors and effector proteins which otherwise are important for activation of intracellular signaling pathways, such as RAS/ERK, phosphatidylinositol 3-kinase/AKT, and Jun-associated N-terminal kinase pathways [129] | Severe defect in the development of enteric nervous system in 40% of cases [129] | |
Homozygous missense mutation (CGG to TGG) at codon 969 of RET with amino acid change from arginine to tryptophan [130] | Critical alteration in RET tyrosine kinase activity [130] | Total gastrointestinal tract aganglionosis [130] | |
Heterozygous RET mutation [128] | C620R mutation [128] | - | Hirschsprung's disease including hypoganglionosis of gastrointestinal tract [128] |
Mutations | Domains affected | Exons affected | Introns affected | Codons affected | Outcomes |
Germline mutation [95],[96] | Extracellular domain [95],[96] | 10 | - | 609, 611, 618, 620 | Hirschsprung's disease |
11 | - | 630, 634 | |||
Intracellular domain-tyrosine kinase 1 residue of tyrosine kinase domain [95],[96] | 15 | - | 883, 891 | ||
14 | - | 804 | |||
15 | - | 883, 891, 918 | |||
Germline mutation [97] | - | 10 | - | c.1852 T>C |
Mutations | Domains Affected | Exons affected | Introns affected | Codons affected | Outcomes |
Nonsense mutation | Extracellular domain [98] | 2, 3, 4, 5, 6 [98] | - | - | Hirschsprung's disease |
Amino acid substitution [98]–[101] | - | - | - | Familial or sporadic cases of Hirschsprung's disease | |
Frameshift mutation | RET gene | - | - | Phe147del [102] | Hirschsprung's disease |
Amino acid substitution in protein truncation of RET [98]–[101] | - | - | - | Familial or sporadic cases of Hirschsprung's disease | |
Point mutation | RET gene in heterozygous state [24],[98],[103] | - | - | - | Hirschsprung's disease |
Mutations | Domains Affected | Exons affected | Introns affected | Codons affected | Outcomes |
Missense mutation | Extracellular domain [98] | 2, 3, 4, 5, 6 [98] | - | - | Hirschsprung's disease |
Impair the RET kinase activity leading to the impairment of the phospholipase C-γ signaling pathway [104] | - | - | E762Q, S767R, R972G, M900T [104] | ||
Complete loss of RET kinase activity [104] | - | - | S765P, R873Q, F893L, R897Q, E921K [104] | ||
RET tyrosine kinase domain [23] | - | - | - | ||
Dominant negative effect through loss of function [24],[105],[106] | - | - | - | ||
- | 15 [107] | - | At nucleotide 2813G to A with R873Q exchange in codon 873 [107] | ||
3 [108] | - | Nucleotide change GTG to ATG (V202M mutation) [108] | |||
7 [108] | - | Nucleotide change GAA to AAA (E480K mutation) [108] | Rectosigmoidal aganglionosis | ||
17 [108] | - | Nucleotide change CCA to ATA (P973L mutation) [108] | |||
13 [108] | - | Nucleotide change GAC to AAC (D77/N mutation) [108] | Total gut wall aganglionosis | ||
Amino acid substitution in RET protein [98]–[101] | - | - | - | Familial or sporadic cases of Hirschsprung's disease |
Mutations | Locations | Outcomes |
Deletion [103] | RET gene [103] | Hirschsprung's disease In 20% patient have low efficiency in detection of deletion [103] |
Partial deletion [109] | RET locus at pericentromeric region of chromosome 10 [109] | |
Interstitial deletion [110] | In the long arm of chromosome 10- del10(q11.21, q21.2) [110] | Total colonic aganglionosis and minor involvement of myenteric plexus [110] |
Proximal deletion [111] | In the long arm 10- del10q11.2 to q21.2 Deletion location likely lying between loci D10S208 and D10S196 [111] |
Colonic aganglionosis in hindgut [111]. |
Cytogenetic deletion [112] | del (10) (q11.2 to q21.2) [112] | Total aganglionosis with small bowel involvement [112] |
Mutations | Locations | Effects | Outcomes |
Mutation in extracellular domain | N terminus region of RET protein [113]–[115] | Affect the amino acid residue No glycosylation of immature 150-kDa form in the endoplasmic reticulum No production of mature 170k-Da form of RET protein No expression of RET protein in cell membrane [113],[114] |
Hirschsprung's disease [113]–[115] |
Mutations | Locations | Effects | Outcomes |
Mutation in intracellular domain | Tyrosine kinase domain [23] | Impaired intracellular signaling pathways [23] | Hirschsprung's disease |
Tyrosine kinase domain 1 [Glu 762-Gln (E762Q), Ser65 to Pro (S765P) and Ser767 to Arg (S767R)] or tyrosine kinase domain 2 [Arg 873 to Gln (R897Q), Glu 921 to Lys (E921K), Arg 972 to Gly (R972G), Pro 973 to Leu (P973L) and Met 980 to Thr (M980T)] [24],[98]–[100],[116]–[119] | - | Familial and sporadic Hirschsprung's disease [24],[98]–[100],[116]–[119] | |
Tyrosine residue at position 1062 which is intracytoplasmic docking site of RET protein [120] | Impaired fixation of SHc to RET protein and thus prevention of the phosphorylation and inhibition of the signaling pathway and thus exert negative effect in the enteric neurogenesis [120] | Hirschsprung's disease |
Expression of RET gene | Effects | Outcomes |
Insufficient level of expression | Insufficient expression of RET protein on the cell surface for GDNF and its co-receptor GFR α1–4 [101],[121] | Hirschsprung's disease |
Mutations | Locations | Effects | Outcomes |
Mutation in exons | Exon 2 in codon 32 changing CTG to TTG [98] | Changes the protein sequence of extracellular domain of RET from serine to leucine [98] | Congenital absence of enteric neurons and ganglia in intestine [98] |
Exon 3 in codon 180 changing CGA to TGA [98] | Changes the protein sequence of extracellular domain of RET from arginine to stop codon [98]. | ||
Exon 5 in codon 330 changing CGG to CAG [98] | Changes the protein sequence in extracellular domain of RET from arginine to glutamine [98] | ||
Exon 6 in codon 393 changing TTC to TTA [98] | Changes the protein sequence of extracellular domain of RET from phenylalanine to leucine [98] | ||
Exon 10 with nucleotide change of C1876A and amino acid change of Q6226K [122] | - | Sporadic ultra-short-segment aganglionosis [122] | |
Exon 11 with nucleotide change of C1941T and amino acid change of 16471 [122] | - | Sporadic long-segment aganglionosis [122] | |
Exon 10 with change in five cysteine codons from Cys to Trp at codon 699 and Cys to Arg at codon 618 or 620 [97] | - | Hirschsprung's disease | |
Exon 2 with change in nucleotide from C254 G to A [123] | Loss of function of RET gene [123] | Total colonic aganglionosis [123] | |
Exon 13 with change in nucleotide from C2308 C to T [123] | |||
Exon 14 with change in nucleotide from C2578 C to T [123] | |||
Exon 4 with change in nucleotide from C789 C to G [123] | - | Long segment Hirschsprung's [123] |
Mutations | Effects | Outcomes |
RET isoforms mutations and defects | RET9 [124] | Lack of enteric ganglion in colon [124] |
Mutation of tyrosine 1062 of RET9 to phenylalanine [125] | Deficient in enteric nervous system [125] |
Mutations | Locations | Effects | Outcomes |
Mutation of enhancer [126] | Enhancer domain of the RET gene in intron 1 (CrS2435357) [126] | - | Hirschsprung's disease with significantly higher impact in males than females [126] |
Promoter defect [127] | Methylation of promoter of RET has 5′ CC-3′ [127] | - | Colonic aganglionosis [127] |
Mutation in introns | Alteration in intron 4 at putative branch site of 24 nucleotides in front of exon 15 with nucleotide exchange of G to A [107] | - | Hirschsprung's disease [107] |
Missense mutation in intron 19 (IVS 19-19 C/T) [108] | - | Only rectosigmoidal aganglionosis [108] |
Mutations | Locations | Effects | Outcomes |
Homozygous RET mutations | C620R mutation [128] | - | Hirschsprung's diseases [128] |
Mutation of tyrosine 1062 in RET with phenylalanine [129] | Impairing the binding site of tyrosine 1062 for phosphotyrosine-binding domains for several adaptors and effector proteins which otherwise are important for activation of intracellular signaling pathways, such as RAS/ERK, phosphatidylinositol 3-kinase/AKT, and Jun-associated N-terminal kinase pathways [129] | Severe defect in the development of enteric nervous system in 40% of cases [129] | |
Homozygous missense mutation (CGG to TGG) at codon 969 of RET with amino acid change from arginine to tryptophan [130] | Critical alteration in RET tyrosine kinase activity [130] | Total gastrointestinal tract aganglionosis [130] | |
Heterozygous RET mutation [128] | C620R mutation [128] | - | Hirschsprung's disease including hypoganglionosis of gastrointestinal tract [128] |