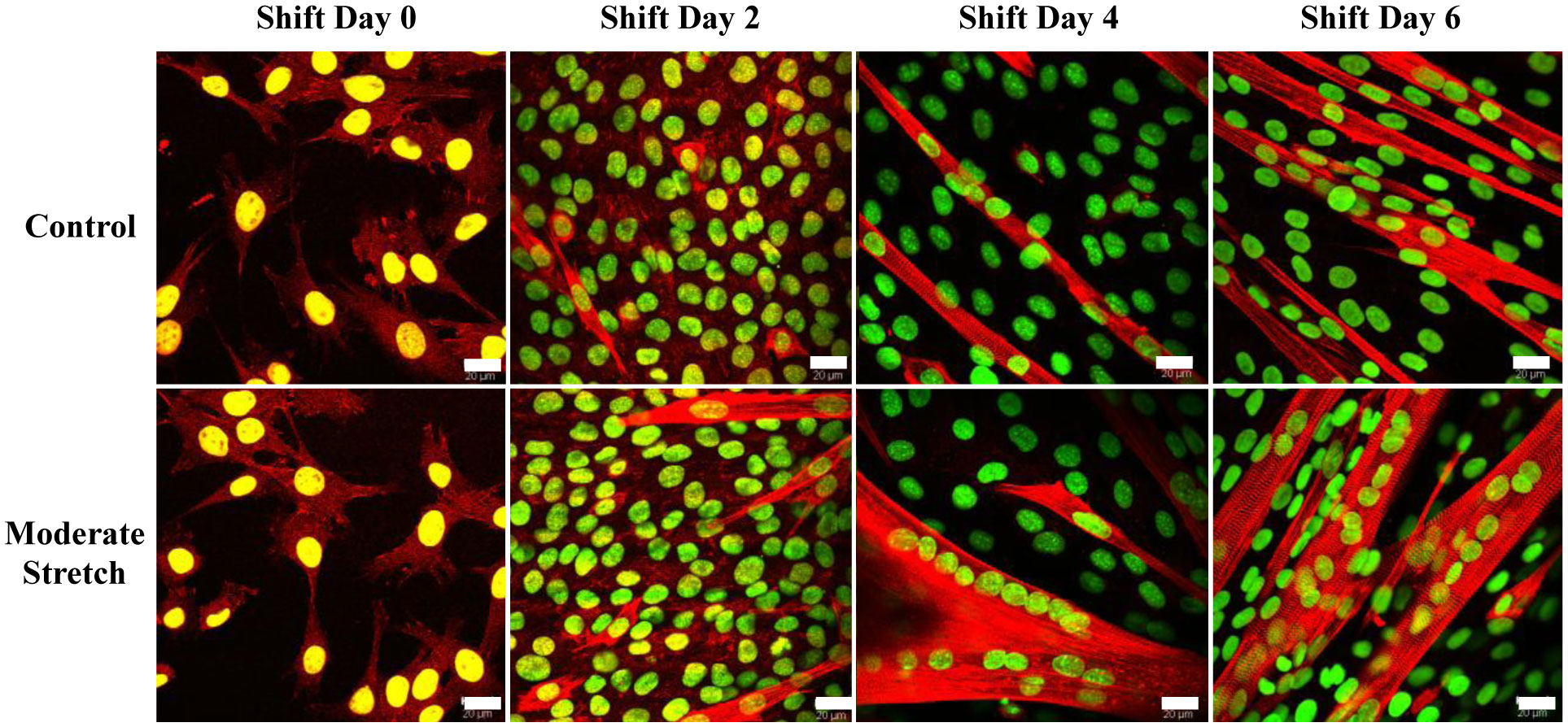
We examined whether mechanical stretch affected expression of muscle-specific microRNAs (miRNAs) that regulate proliferation (miR-133a) or differentiation (miR-1, -206). Real-time quantitative RT-PCR was used to assess miRNA regulation in the murine myoblast cell line C2C12 exposed to stretch regimens that promote either proliferation (high stretch: 17%, 1 Hz) or differentiation (moderate stretch: 10%, 0.5 Hz) after adding media that promotes differentiation. Controls consisted of myoblasts cultured under static conditions. While miRNA expression was not affected by high stretch, a significant effect of stretch (P < 0.05) was seen after 4 days with the moderate stretch regimen. All three microRNAs were upregulated by stretch, with the most significant increase for miR-1. Myoblast maturation was enhanced with a moderate stretch regimen, as assessed by a higher percentage of nuclei in straited fibers and an increase in Mef2c gene expression. Correspondingly, HDAC4 protein expression, a direct target of miR-1 and repressor of Mef2, was decreased with the moderate stretch regimen. Over-expression of miR-1 abrogated the effect of stretch on miR-1, miR-133a and miR-206 levels compared to its negative control but did not alter miR-133a or miR-206 levels. Treatment with an antisense mRNA to miR-1 similarly diminished the stretch-mediated response. Results indicate that the differential response of skeletal myoblasts to moderate and high stretch cyclic stretch regimens is due, in part, to muscle specific miRNA expression.
Citation: Caroline Rhim, William E. Kraus, George A. Truskey. Biomechanical effects on microRNA expression in skeletal muscle differentiation[J]. AIMS Bioengineering, 2020, 7(3): 147-164. doi: 10.3934/bioeng.2020014
We examined whether mechanical stretch affected expression of muscle-specific microRNAs (miRNAs) that regulate proliferation (miR-133a) or differentiation (miR-1, -206). Real-time quantitative RT-PCR was used to assess miRNA regulation in the murine myoblast cell line C2C12 exposed to stretch regimens that promote either proliferation (high stretch: 17%, 1 Hz) or differentiation (moderate stretch: 10%, 0.5 Hz) after adding media that promotes differentiation. Controls consisted of myoblasts cultured under static conditions. While miRNA expression was not affected by high stretch, a significant effect of stretch (P < 0.05) was seen after 4 days with the moderate stretch regimen. All three microRNAs were upregulated by stretch, with the most significant increase for miR-1. Myoblast maturation was enhanced with a moderate stretch regimen, as assessed by a higher percentage of nuclei in straited fibers and an increase in Mef2c gene expression. Correspondingly, HDAC4 protein expression, a direct target of miR-1 and repressor of Mef2, was decreased with the moderate stretch regimen. Over-expression of miR-1 abrogated the effect of stretch on miR-1, miR-133a and miR-206 levels compared to its negative control but did not alter miR-133a or miR-206 levels. Treatment with an antisense mRNA to miR-1 similarly diminished the stretch-mediated response. Results indicate that the differential response of skeletal myoblasts to moderate and high stretch cyclic stretch regimens is due, in part, to muscle specific miRNA expression.
Skeletal myoblasts are sensitive to passive mechanical stimulation. The normal range for muscle stretch is quite narrow (10–20%), and stretch beyond this point leads to muscle injury [1]. Application of 10% stretch at moderate frequencies (0.5 Hz) with longer rest periods enhanced myoblast fusion and differentiation [2]. When skeletal muscle myoblasts are stretched at low frequencies (<0.5 Hz) and high intensities (>15% stretch) [3],[4], mechanical stretch results in increased protein synthesis and cellular hypertrophy, while a higher frequency (>1 Hz) and low intensity (<5% stretch) regimen causes an increase in metabolic activity, without major changes to muscle morphology [3]. In contrast, a 17%-stretch applied over a 1-hour stretch cycle at 1 Hz followed by a 23-hour rest period inhibited myogenesis as the myoblasts were directed toward a more proliferative response through increased gene expression of various factors, including cyclin D1 [5]. While few studies have demonstrated that the pro-myogenic factor, myocyte enhancer factor 2 (Mef2), and its repressor, histone deacetylase 4 (HDAC4), are affected by mechanical stimulation directly, key mechano-sensitive pathways, such as calcium/calmodulin [6] and MAPK signal transduction pathways [7], are associated with both proteins [8]–[11].
MicroRNAs (or miRNAs), or short non-coding segments of RNA, ∼20–22 nucleotides in length, regulate expression of mRNAs which encode key proteins that influence proliferation or differentiation in skeletal muscle [12]–[14]. Three miRNAs involved in myogenesis are miR-1, miR-133a, and miR-206 [15]–[18]. MiR-1 and miR-133a promote skeletal muscle differentiation and proliferation, respectively [12]. Among its many targets, miR-1 targets HDAC4, which is a transcriptional repressor of Mef2, which is critical for myoblast differentiation [19]. Similarly, miR-133a down-regulates serum response factor (SRF), which blocks myoblast proliferation. Although the miR-1 and miR-133a genes are transcribed together and controlled at transcription, they regulate protein expression of two different pathways that work in a complementary manner in the muscle maturation process. Both knockdown via antisense oligonucleotide probes and over-expression studies confirmed that miR-1 plays a critical role in myogenesis while miR-133a alone promoted proliferation and partially inhibited myoblast differentiation [12],[20]. Exclusive to skeletal muscle [21], differentiation is further enhanced by miR-206, and may influence hypertrophy and fiber type transition. MiR-206 down-regulates DNA polymerase α (Pol α1), which led to cell cycle arrest and eventual cell differentiation [14] and miR-206 expression was elevated in newly formed myotubes [22].
Skeletal muscle microRNAs appear to be sensitive to mechanical stimulation. We previously found that the muscle specific miRs, miR-1 and miR-133a increased in human skeletal muscle myoblasts after exposure to the moderate stretch regimen for two weeks in 8% serum differentiation medium but decreased in response to stretch when the medium had 2% serum [23]. Downstream changes to miR targets were not investigated. Continuous cyclic stretch (0.17 Hz, 5% stretch for 48 hours) upregulated miR-146a, which affected levels of its target, Numb, and promoted proliferation inhibited myogenesis and an anti-miR to miR-146 blocked strength-induced proliferation and led to myoblast differentiation [24]. Levels of miR-1 and 133a are downregulated in muscle hypertrophy models [25]. Further, validated targets for key muscle-specific miRNAs (both skeletal and cardiac), such as SRF, RhoA, and cdc42, are also crucial in signaling pathways associated with mechanical stimulation [12],[26],[27].
In this study we examined the hypothesis that miRNAs respond in a differential manner to moderate (10%) and high (17%) stretch regimens in a manner consistent with the extent of myoblast differentiation to muscle fibers under these stretch conditions. We examined the mouse C2C12 cell line, since the muscle-specific microRNA levels were higher in the mouse than human myoblasts [23]. Because miRNAs can modulate specific functions of differentiated skeletal muscle, they represent a potential target for manipulation and may be enhanced by mechanical stimulation.
Murine C2C12 myoblasts (ATCC, Rockville, MD), a subclone derived from a cell line that originated from normal adult C3H mouse leg muscle [28], were grown on a 6-well tissue culture plate (Flexcell International, Hillsborough, NC) coated with 100 µg/ml growth factor reduced Matrigel® (GFR Matrigel, BD Biosciences, Bedford, MA). The cells were fed daily with growth medium (GM) containing high glucose Dulbecco's modified Eagles' medium (DMEM; Gibco/Invitrogen, Carlsbad, CA), 8% new born calf serum (HyClone Laboratories, Logan, UT), 8% fetal bovine serum (HyClone), 0.5% chicken embryo extract (Accurate Chemicals, Westbury, NY), and 0.1% gentamicin (Gibco/Invitrogen) at 37 °C and 5% CO2 until the cells reached 85–90% confluency. To promote differentiation of myoblasts to fuse into myotubes, the growth medium was shifted to differentiation medium (DM), consisting of DMEM supplemented with 8% horse serum (HyClone) and 0.1% gentamicin (Gibco/Invitrogen).
Mechanical stretch was applied using a FT-4000T Flexercell Unit (Flexcell International). After culturing the C2C12 myoblasts on silastic membrane 6-well plates (Flexcell International) for approximately 2 days and allowing them to reach 85–90% confluency, the GM was changed to DM and one of two mechanical regimens was started. The moderate stretch regimen consisted of a cyclic stretch at 0.5 Hz, 10% stretch for 1-hour with a 5-hour rest period [2]. The high stretch regimen consisted of a cyclic stretch at 1 Hz, 17% stretch for 1-hour with a 23-hour rest period [5]. Each regimen was sustained for up to 6 days.
Total RNA was isolated and purified using the mirVana miRNA Isolation Kit (Ambion). RNA concentration and RNA quality were measured and assessed using the NanoDrop ND-1000 spectrophotometer (NanoDrop, Wilmington, DE). cDNA was prepared using the microRNA reverse transcriptase kit (ABI, Applied Biosystems, Foster City, CA) with a MyCycler (Bio-Rad) thermal cycler per manufacturer instructions. The TaqMan Universal PCR Master Mix (Applied Biosystems) was combined with the cDNA samples and the resulting solutions were placed in the 7300HT Real-Time PCR Detection System (ABI) using standard kit protocols. The 2−ΔΔCT method was used to analyze the real-time gene expression data [29]. The appropriate hairpin primers were included in the TaqMan miRNA RT-PCR assays that were purchased for each miRNA of interest. A small nucleolar RNA (RNU6B, ABI) was used as the endogenous control, and RNA isolated from mouse embryo (10–12 days old) was used as a calibrator. For the muscle-specific genes of interest, SYBR green chemistry was used and melt curve analyses were done for each reaction to ensure that the desired amplification was completed. Oligonucleotide primers were obtained from HS Kim (UNC) and IDT Technologies.
A 10 µg/µl sample of total RNA was processed by the Duke University Microarray Facility using the mirVana miRNA labeling kit and miRNA probe set (Ambion and Invitrogen sets), which was used to end label and purify small RNA probes and markers. Three separate microarray studies were completed. In the first microarray experiment, cells of passage six were cultured under static conditions and the extracted total RNA (including miRNAs) were labeled with a Cy3 dye. Total RNA from the stretched cells were Cy5-labeled. In the second and third microarray experiments, the unstretched and stretched miRNA samples were Cy5-labeled while miRNAs from normal mouse embryo (10–12 days) tissue (Ambion First Choice Total RNA) were labeled with a Cy3 dye. The fluorescence intensities of these two dyes reflect the levels of a given miRNA, which is tracked by the hybridization location. The results from the first were presented as output ratios of Cy5:Cy3 fluorescence, signifying the stretched to unstretched miRNA ratios. In the second and third studies, the Cy5:Cy3 ratios were obtained separately for both the unstretched control and stretched RNA samples, then, these ratios were compared to each other. This method filters noise more efficiently, but manual filtering of all three microarray studies was sufficient to give reliable results. Each microarray chip consisted of over 662 probes, with a majority from human, mouse, and rat species (Ambion, Invitrogen). RNA purity analysis was completed using the NanoDrop ND-1000 spectrophotometer (NanoDrop) and the Agilent Bioanalyzer (Agilent, San Jose, CA).
Skeletal myoblast cultures were fixed with 3.7% formaldehyde for 15 minutes at room temperature and permeabilized in 0.5% Triton-X (Sigma, St. Louis, MO) in PBS for 15 minutes. Cells were then incubated with 10% goat serum in PBS for 30 minutes at 37 °C to block non-specific binding and incubated in PBS for 1 hour with specific antibodies (α-actinin, Sigma). The primary antibody was detected with a secondary mouse anti-IgG conjugated with Alexa Fluor 546-labeled secondary antibody (Invitrogen). Sytox Green (Invitrogen) was added after the secondary incubation as a counter stain for nuclei. Fluorescent images were obtained using an inverted fluorescent microscope (Zeiss Axiovert S-100, Carl Zeiss Inc.) connected to a digital camera (Carl Zeiss Inc.). The percentage of nuclei in striated fibers was determined by counting the number of nuclei directly associated with α-actinin stained myofibers and dividing by the total number of nuclei.
Cultured C2C12 cells were lysed in a 1:10 solution of Cell-Lytic M and protease/phosphatase inhibitor (Sigma) for approximately 30 min on ice. Nuclear and insoluble debris are removed by centrifuging for 5 min at 10000 rpm. Protein concentrations were determined by the BCA assay (Pierce/Thermo Scientific, Rockford, IL) and at least 20 µg of solubilized total protein were loaded in each lane of a 10% (w/v) Tris-HCl polyacrylamide gel (Bio-Rad, Hercules, CA). Transfer of the protein to a PVDF membrane (BioRad) was completed overnight, and the membrane was blocked with 5% nonfat dry milk in TBST (Tris-buffered saline with 0.1% Tween 20) for 1 hour at room temperature. The membranes were then incubated with the primary antibodies overnight at 4 °C in a 1% nonfat dry milk-TBST solution. Primary antibodies were obtained from the following resources: GAPDH (Abcam, Cambridge, MA), PCNA, α-actinin, HDAC4 (Santa Cruz Biotechnology, Santa Cruz, CA). After rinsing with TBST, the membranes were incubated with a horseradish peroxidase-conjugated IgG anti-mouse or anti-rabbit antibody (SCBT) for 1 hour at room temperature. Proteins were detected by ECL SuperSignal West (Pierce/Thermo Scientific) on blue X-ray films. Developed films were scanned and subjected to densitometry measurement using NIH Image J (Version 1.62) and expressed as intensity in arbitrary units. At least three independent experiments were used for each condition.
For the over-expression studies, Pre-miR precursor molecules specific for the mature miR-1 sequence (Ambion) were transfected with siPORT NeoFX transfection agent (Ambion) into myoblasts per manufacturer instructions. A Cy-3 labeled, non-targeting random sequence negative control (Pre-miR Negative Control #1, Ambion) was used to obtain transfection efficiencies. Briefly, the transfection agent was diluted in Opti-MEM I (Gibco) and mixed with 50 nM of the Pre-miR-1 precursors or with the same concentration of the negative control. After incubating for 10 minutes at room temperature, the mixture was then dispensed into the appropriate wells. The cell suspension was added to the wells, and cultured in normal conditions for 24 hours, with antibiotic-free GM. The GM was then replaced with fresh DM, at which point cell viability and morphology were assessed, and if normal, the moderate stretch regimen was started. Proper transfection was verified using qRT-PCR to verify that the miRNA was expressed in the mature form at higher levels. The same transfection procedures outlined above were used with Anti-miR-1 oligonucleotides (Ambion) and the corresponding Anti-miR random sequence negative control (Ambion). Treatment with an antisense mRNA to miR-1 was assessed via Western blot analysis of its target, HDAC4.
Data are presented as a mean ± standard error (SEM) unless otherwise noted. A two-factor ANOVA or student t-tests were performed using the Statview 5.0 statistical analysis package. When applicable, the Bonferroni post-hoc test was performed as part of the ANOVA analysis. A value of p < 0.05 was considered significant unless otherwise noted. Comparison of regression slopes for stretch and control were used to assess the effect of stretch on miR levels [30].
We examined the response of C2C12 myoblasts to two stretch regimens: a cyclic stretch regimen of 0.5 Hz, 10% stretch for 1 hour with a 5-hour rest period, which promotes differentiation [2] and a 1.0 Hz, 17% stretch for 1 hour with a 23-hour rest period, which promotes proliferation [5].
Immunostaining with α-actinin at various time points showed a differential response in myoblast morphology with the two stretch regimens. Multinucleated myotubes and striations were still apparent in the unstretched control but were not as clearly organized as those subjected to a moderate stretch regimen from shift day 0 to shift day 6 (Figure 1). Between shift day 0 and shift day 2, myoblasts have not all exited the cell cycle and continue to proliferate, as confirmed by the nuclei staining showing a notable increase in cell number by shift day 2, compared to shift day 0. By shift day 4, the effect of stretch became apparent with the appearance of more multinucleation and fiber formation. Myotubes often formed on top of a confluent layer of myoblasts, which explains the seemingly lower number of nuclei from shift day 2 to shift day 4 but is more an attribute of the method of imaging. At shift day 6, striations were clearly visible in the stretched samples. On the other hand, there was less multinucleation and fiber formation in the myoblasts subjected to the high stretch (17%) regimen compared to its unstretched control (Figure 2).
The percentage of nuclei in striated fibers was used to quantify myofiber differentiation (Table 1). A myogenic switch occurred approximately 4 days after shifting the media, at shift day 4, which corresponds with myoblast morphology (see Figure 1) confirming a marked increase in myotube formation at this time point. An ANOVA showed a significant effect of time (p < 0.001), however, only the moderate stretch regimen produced a significant effect of stretch (p < 0.02). A significant difference in the percentage of nuclei in striated fibers was evident with the moderate stretch regimen at shift day 4, but there was no difference by shift day 6. Conversely, there was no difference in the percentage of nuclei in striated fibers between unstretched and stretched samples at any time point when subjected to a high stretch regimen. There was a significant difference in the percentage of nuclei in striated fibers for those cells subjected to the moderate stretch regimen compared to the high stretch regimen by shift day 6 where an ANOVA with all data combined from each regimen showed a significant effect of stretch (moderate or high stretch) regimen (p < 0.02). Thus, the moderate stretch regimen produced an increase in differentiation relative to unstretched controls or the high stretch conditions.
Moderate Stretch Ratio (n = 7) | High Stretch Ratio (n = 5) | |||
Control | Stretch | Control | Stretch | |
Shift Day 2 | 0.5 ± 0.3 | 0.9 ± 0.5 | 0.7 ± 0.2 | 1.3 ± 0.4 |
Shift Day 4 | 30.8 ± 1.6* | 42.7 ± 4.3* | 28.7 ± 4.5 | 31.2 ± 4.6 |
Shift Day 6 | 47.9 ± 3.0 | 52.1 ± 3.8# | 47.5 ± 3.8 | 39.0 ± 5.3# |
*p < 0.05, Control vs. Stretch; #p < 0.05, Moderate Strain vs. High Stretch Ratio
n = number of independent experiments.
A microRNA array analysis of myofibers undergoing the moderate stretch regimen for 4 days and unstretched controls indicated that the following microRNAs exhibited at least a 40% increase in at least one of the 3 microarrays: miR-31, miR-125b, miR-133a, miR-140, miR-206, miR-214, and miR-422b (Table S1). miR-1 was not part of the array, but two other muscle-specific microRNAs, miR-133a and miR-206 were sensitive to the moderate stretch regimen.
To assess the effect of stretch over time, C2C12 myoblasts were placed in differentiation medium and exposed to the moderate stretch regiment, the high stretch regimen or the unstretched control case and levels of microRNAs were determined by qRT-PCR. To show the wide range in miR expression throughout differentiation, we also assessed the baseline expression levels of miR-1, 133a, and 206 over time in the absence of stretch, and observed marked increases in all three miRNAs relative to shift day 0 (Supplementary Figure S1). All three miRNAs were in low abundance at shift day 0. Levels of miR-1 and miR-133a increased on a similar scale while miR-206 was present at significantly lower levels. Expression levels of miR-1, 133a, and 206 from both unstretched and stretched samples were fit to a linear regression over 6 days after shifting to a reduced growth factor serum media. The moderate stretch regimen produced a significant increase in slope (Figure 3), where miR-1 and 133a levels were up-regulated with stretch at an earlier time point than miR-206 (shift day 3 vs. shift day 4) (dashed vertical lines). There were no significant differences between stretched and unstretched cells with the high stretch (17%) regimen.
Next, we examined the effect of the moderate stretch regimen on the other microRNAs identified by the microarray analysis as being stretch sensitive: miR-31, miR-125b, miR-140, miR-214, and miR-422b (Supplementary Figures S2 and S3). miR-214 [31] and miR-422b (also known as miR-378) [32] promote myoblast differentiation, miR-140 downregulates WNT11 and promotes hypertrophy and decreases lactic acid production [33], miR-31 promotes satellite cell proliferation [34], and miR-125b is downregulated during myoblast differentiation [35]. All of these microRNAs are found in skeletal muscle. Only miR-422b increased monotonically with time. The others increased over the first 4–6 days and then declined. None of these microRNAs showed a difference between the moderate strain regimen and unstretched controls.
Because miR-1, mir-133a and miR-206 affect specific mRNA targets, we examined baseline changes in several muscle-specific genes, which included key regulators MyoD, myogenin, Mef2c, and SRF. The gene expression of validated targets of miR-1 (HDAC4) and miR-133a (SRF) was also examined to assess whether the changes in miRNA levels induced by stretch were linked to changes in the levels of these genes. Neither the moderate nor high stretch regimens significantly affected MyoD as a global ANOVA showed no effect of time or stretch regimen (Supplementary Figure 4A). There was a significant effect of time (p < 0.0002) and stretch regimen (p < 0.02) on myogenin gene expression (Supplementary Figure S4B), and the post hoc test only detected significant differences between the moderate and high stretch regimens on day 2 (p < 0.05). Similarly, there was also an effect of time (p < 0.0001) and regimen (p < 0.02) on HDAC4 gene expression, but the post hoc test did not find significance (p < 0.05) between the control and moderate or high stretch regimen samples at any given time point. SRF gene expression was unchanged with time or regimen.
In contrast, levels of Mef2c mRNA were greater in myoblasts subjected to the moderate stretch regimen by shift day 6 (Figure 4). Relative to unstretched control, Mef2c levels were unchanged in myoblasts stretched at high stretch. A two-factor ANOVA verified a significant effect of time (p < 0.001) and regimen (control vs. moderate vs. high stretch) (p < 0.01). A Bonferroni post hoc test showed significance between Mef2c expression in unstretched controls and the moderate stretch samples on days 4 and 6 as well as between the moderate and high stretch samples on days 4 and 6. There was no significant difference between the unstretched controls and the high stretch samples over the entire temporal profile.
These gene expression results suggest that observed changes in the three muscle-specific miRNAs did not alter several critical mRNA levels. However, since miRNAs are translational regulators, we examined the protein levels of the miRNA target, HDAC4, which is a downstream target of miR-1 and effector of Mef2. Western blot analysis indicated a decreasing trend in HDAC4 protein expression in myoblasts exposed to a moderate stretch cyclic stretch regimen (Figure 5). A two-factor ANOVA found significant effects of time (p < 0.0005) and stretch (p < 0.02), but no interaction between the two. A Bonferroni correction showed that moderate stretch was less than control levels at shift days 2 and 6 (p < 0.05). There was no effect of stretch on HDAC4 protein levels with the high stretch regimen. Although there were no major differences in HDAC4 levels with stretch (moderate or high stretch) on the transcriptional level, this change on the translational level with the moderate stretch regimen suggested that as miR-1 levels are upregulated by stretch four days after shifting to DM, the downstream effect of suppressing HDAC4 protein levels was more pronounced at later time points.
The effect of stretch was also examined in conjunction with the over-expression of one of the myogenic miRNAs, miR-1, which promotes myoblast differentiation. Results shown in Figure 6 indicated that miR-1 levels were indeed over-expressed via transient transfection with Pre-miR-1 precursor molecules, as assessed by qRT-PCR, there was an increase in miR-1 levels over four orders of magnitude one day after transfection, or at shift day 0 (p < 0.0001), but these levels were drastically reduced by shift day 2 (p = 0.064). Levels of miR-1 were nearly equal to those of the negative control by shift day 4.
In order to assess whether the effects of stretching myoblasts are affected by miRNA over-expression, we examined changes in miRNA levels with miR-1 over-expression combined with stretch (Figure 7). Results represent the fold change relative to unstretched cells. The negative controls consisted of a scrambled miR sequence. At shift day 2, qRT-PCR showed a small, but significant down-regulation of miR-1 levels when stretch was combined with miR-1 over-expression. After 4 days of stretch, the relative change in miR-1 was similar to what was observed with the negative control. The change in levels of miR-133a and miR-206 with miR-1 over-expression and stretch was not different from their negative controls, verifying that over-expression of one miRNA did not have major effects on the other microRNA levels.
Since blocking key muscle-specific miRNAs (particularly miR-1) is detrimental to promoting myoblast differentiation [12], we hypothesized that stretch might compensate for the transient knockdown of miR-1, but the results show that miR-1 may be critical for a stretch-mediated effect to occur. Since anti-miR-1 oligonucleotides do not degrade the mature miR-1 sequence, qRT-PCR could not be used to assess the degree of inhibition. Therefore, the effect of inhibiting miR-1 was assessed by HDAC4 protein expression because it is a direct target for miR-1. HDAC4 levels increased slightly, as expected, in anti-miR-1-treated myoblasts. We then plotted the HDAC4 protein levels after anti-miR-1 treatment relative to the HDAC4 protein levels after treatment with the negative control microRNA sequence (Figure 8). A ratio of 1 would indicate that the antimir-1 treatment had no effect since the response is the same as for control conditions. A ratio greater than 1 would indicate that the anti-miR-1 treatment increased HDAC4 protein relative to control conditions. The effect of anti-miR-1 treatment was most apparent at shift day 2 with ratios of anti-miR-1 to negative control HDAC4 protein levels greater than 1 (p < 0.05) in both unstretched and stretched samples (Figure 8). By shift day 4, HDAC4 levels between anti-miR-1 and negative control myoblasts were nearly the same (ratio ∼1), suggesting that miR-1 inhibition was removed by this time. This time scale was similar to that observed with miR-1 over-expression (Figure 6). These results show that anti-miR-1 treatment does increase HDAC4 protein at early times and stretch alone does not compensate for this effect.
Results of this study demonstrated a differential response of skeletal myoblasts to moderate and high cyclic stretch regimens that involved the muscle-specific microRNAs miR-1, miR-133a and miR-206. Myoblast maturation was enhanced with a moderate stretch regimen that increased Mef2c gene expression, although the muscle-specific genes MyoD and myogenin were not affected. The moderate stretch regimen produced a significant increase in in miR-1, 133a, and 206 expression with stretch, a significant effect of stretch (p < 0.05) was seen after 3 or 4 days, post-differentiation. HDAC4 protein expression, a direct target of miR-1 and repressor of Mef2, was decreased with the moderate stretch regimen. Over-expression of miR-1 abrogated the effect of moderate stretch regimen in miR-1 levels and inhibition of miR-1 blocked the stretch-mediated response in HDAC4 protein. These results suggest that the muscle-specific miRNAs are crucial in promoting a response to stretch, however, there is a complex interplay of the effect of mechanical stimulation on downstream regulation of muscle-specific genes and miRNAs.
The significant increase in miR-1, miR-133a and miR-206 levels with differentiation are consistent with previous time course studies [12],[14], and differences in miR-1 and miR-206 levels have been reported among different muscles [36]. Both miR-1 and miR-133a had parallel responses which were consistent with similar regulation of these two miRNAs [12],[13],[37]. Muscle differentiation is further enhanced by miR-206, and blocking it hindered withdrawal from the cell cycle and subsequent fusion and differentiation [14].
Most studies of miR-1, miR-133a, and miR-206 have focused on miRNA dysregulation in cardiac hypertrophy [26],[38]–[40]. In skeletal muscle, the effects of hypertrophy on miR-1, miR-133a, and miR-206 regulation were examined by in vivo injury to rat soleus and plantaris muscles. The level of miR-1 and miR-133a decreased following 7 days of functional overload, and levels of miR-206 did not change [25]. These studies are consistent with a mechanical link to miRNA regulation.
While the effect of stretch produced modest changes in the levels if miR-1, miR-133a and miR-206, a 2.8-fold increase in miR-1 was observed in human hearts with coronary artery disease and could produce conduction abnormalities by reducing expression of connexin 43 and a potassium channel [41]. Similar changes in miR-1 and miR-133a levels in a cardiac hypertrophy model caused changes in the targets [26]. Since the change in miRNA levels induced by stretch were comparable to levels reported to induce functional effects in vivo, the functional changes we observed could be attributable to mechanical stimulation.
Although HDAC4 mRNA was not transcriptionally regulated with mechanical stimulation, HDAC4 protein expression was altered with a moderate stretch regimen (Figure 5). HDAC4 represses Mef2 [42],[43], but is also affected by Mef2 expression via a feedback mechanism involving miR-1. We found an upregulation of Mef2c gene expression levels with a moderate stretch regimen (Figure 4) and corresponding increases in three myogenic miRNA levels (miR-1, 133a, and 206). In particular, Mef2 and SRF gene expression can activate expression of miR-1 and miR-133a in both skeletal and cardiac muscle [13],[44],[45]. Mef2 is thought to cooperate with MyoD to regulate an intragenic enhancer of these miRNAs [44]. Although mechanical stimulation with a moderate stretch regimen did not produce a change in MyoD, myogenin, HDAC4,and SRF gene expression, a microRNA-mediated process does not always produce changes in gene expression, and protein levels may be affected as we observed with HDAC4.
When stretch was combined with miR-1 over-expression, we observed a significant decrease in the stretch-induced effect on the muscle-specific miRs, compared to the negative control. This response may be a control mechanism to keep miRNA levels in balance to prevent gross or permanent inhibition of miR targets [46]. In the miR-1 inhibition experiment, stretch alone was not able to overcome the effect of drastically inhibiting miR-1 (anti-miR-1), when HDAC4 protein expression at early time points (Figure 8). These results imply that the stretch-mediated effect is miR-1 dependent and without a nominal level present, may inhibit other factors that are mechanically linked. Overall, these results support a role for the muscle-specific miRNAs having an important, but modest role in mechanical regulation of skeletal muscle differentiation.
A moderate stretch regimen (10%, 0.5 Hz) produced a modest but significant elevation of the muscle-specific microRNAs miR-1, miR-133a and miR-206. Of these, miR-1 was most significantly affected. A high level of stretch (17%, 1 Hz) did not affect miR levels or promote differentiation, beyond control conditions. Moderate stretch elevated Mef2c mRNA and HDAC4 protein. Addition of anti-miR-1 prevented the reduction in HDAC4 protein levels at early times of differentiation. The results suggest that the muscle specific microRNAs are activated by stretch and are crucial in promoting a response to stretch.
[1] | Burkholder TJ, Lieber RL (2001) Sarcomere length operating range of vertebrate muscles during movement. J Exp Biol 204: 1529-1536. |
[2] |
Zhang SJ, Truskey GA, Kraus WE (2007) Effect of cyclic stretch on β1D-integrin expression and activation of FAK and RhoA. Am J Physiol Cell Physiol 292: C2057-2069. doi: 10.1152/ajpcell.00493.2006
![]() |
[3] | Vandenburgh HH (1992) Mechanical forces and their second messengers in stimulating cell growth in vitro. Am J Physiol 262: R350-R355. |
[4] |
Vandenburgh HH, Karlisch P (1989) Longitudinal growth of skeletal myotubes in vitro in a new horizontal mechanical cell stimulator. In Vitro Cell Dev Biol 25: 607-616. doi: 10.1007/BF02623630
![]() |
[5] |
Kumar A, Murphy R, Robinson P, et al. (2004) Cyclic mechanical strain inhibits skeletal myogenesis through activation of focal adhesion kinase, Rac-1 GTPase, and NF-κB transcription factor. FASEB J 18: 1524-1535. doi: 10.1096/fj.04-2414com
![]() |
[6] |
Rauch C, Loughna PT (2005) Static stretch promotes MEF2A nuclear translocation and expression of neonatal myosin heavy chain in C2C12 myocytes in a calcineurin- and p38-dependent manner. Am J Physiol Cell Physiol 288: C593-C605. doi: 10.1152/ajpcell.00346.2004
![]() |
[7] | Kook SH, Lee HJ, Chung WT, et al. (2008) Cyclic mechanical stretch stimulates the proliferation of C2C12 myoblasts and inhibits their differentiation via prolonged activation of p38 MAPK. Mol Cells 25: 479-486. |
[8] |
Zhao X, Ito A, Kane CD, et al. (2001) The modular nature of histone deacetylase HDAC4 confers phosphorylation-dependent intracellular trafficking. J Biol Chem 276: 35042-35048. doi: 10.1074/jbc.M105086200
![]() |
[9] |
Zhou X, Richon VM, Wang AH, et al. (2000) Histone deacetylase 4 associates with extracellular signal-regulated kinases 1 and 2, and its cellular localization is regulated by oncogenic Ras. Proc Natl Acad Sci USA 97: 14329-14333. doi: 10.1073/pnas.250494697
![]() |
[10] |
McKinsey TA, Zhang CL, Lu J, et al. (2000) Signal-dependent nuclear export of a histone deacetylase regulates muscle differentiation. Nature 408: 106-111. doi: 10.1038/35040593
![]() |
[11] |
Miska EA, Langley E, Wolf D, et al. (2001) Differential localization of HDAC4 orchestrates muscle differentiation. Nucleic Acids Res 29: 3439-3447. doi: 10.1093/nar/29.16.3439
![]() |
[12] |
Chen JF, Mandel EM, Thomson JM, et al. (2006) The role of microRNA-1 and microRNA-133 in skeletal muscle proliferation and differentiation. Nat Genet 38: 228-233. doi: 10.1038/ng1725
![]() |
[13] |
Rao PK, Kumar RM, Farkhondeh M, et al. (2006) Myogenic factors that regulate expression of muscle-specific microRNAs. Proc Natl Acad Sci USA 103: 8721-8726. doi: 10.1073/pnas.0602831103
![]() |
[14] |
Kim HK, Lee YS, Sivaprasad U, et al. (2006) Muscle-specific microRNA miR-206 promotes muscle differentiation. J Cell Biol 174: 677-687. doi: 10.1083/jcb.200603008
![]() |
[15] |
Baskerville S, Bartel DP (2005) Microarray profiling of microRNAs reveals frequent coexpression with neighboring miRNAs and host genes. RNA 11: 241-247. doi: 10.1261/rna.7240905
![]() |
[16] |
Wienholds E, Plasterk RHA (2005) MicroRNA function in animal development. FEBS Lett 579: 5911-5922. doi: 10.1016/j.febslet.2005.07.070
![]() |
[17] |
Lagos-Quintana M, Rauhut R, Yalcin A, et al. (2002) Identification of tissue-specific microRNAs from mouse. Curr Biol 12: 735-739. doi: 10.1016/S0960-9822(02)00809-6
![]() |
[18] |
Sempere LF, Freemantle S, Pitha-Rowe I, et al. (2004) Expression profiling of mammalian microRNAs uncovers a subset of brain-expressed microRNAs with possible roles in murine and human neuronal differentiation. Genome Biol 5: R13. doi: 10.1186/gb-2004-5-3-r13
![]() |
[19] |
Ma K, Chan JKL, Zhu G, et al. (2005) Myocyte enhancer factor 2 acetylation by p300 enhances its DNA binding activity, transcriptional activity, and myogenic differentiation. Mol Cell Biol 25: 3575-3582. doi: 10.1128/MCB.25.9.3575-3582.2005
![]() |
[20] |
Wang DZ (2006) Micro or mega: how important are MicroRNAs in muscle? Cell Cycle 5: 1015-1016. doi: 10.4161/cc.5.10.2742
![]() |
[21] |
McCarthy JJ (2008) MicroRNA-206: the skeletal muscle-specific myomiR. Biochim Biophys Acta 1779: 682-691. doi: 10.1016/j.bbagrm.2008.03.001
![]() |
[22] |
Yuasa K, Hagiwara Y, Ando M, et al. (2008) MicroRNA-206 is highly expressed in newly formed muscle fibers: implications regarding potential for muscle regeneration and maturation in muscular dystrophy. Cell Struct Funct 33: 163-169. doi: 10.1247/csf.08022
![]() |
[23] |
Cheng CS, El-Abd Y, Bui K, et al. (2014) Conditions that promote primary human skeletal myoblast culture and muscle differentiation in vitro. Am J Physiol Cell Physiol 306: C385-C395. doi: 10.1152/ajpcell.00179.2013
![]() |
[24] |
Kuang W, Tan J, Duan Y, et al. (2009) Cyclic stretch induced miR-146a upregulation delays C2C12 myogenic differentiation through inhibition of Numb. Biochem Bioph Res Commun 378: 259-263. doi: 10.1016/j.bbrc.2008.11.041
![]() |
[25] |
McCarthy JJ, Esser KA (2007) MicroRNA-1 and microRNA-133a expression are decreased during skeletal muscle hypertrophy. J Appl Physiol 102: 306-313. doi: 10.1152/japplphysiol.00932.2006
![]() |
[26] |
Care A, Catalucci D, Felicetti F, et al. (2007) MicroRNA-133 controls cardiac hypertrophy. Nat Med 13: 613-618. doi: 10.1038/nm1582
![]() |
[27] |
Carson JA, Wei L (2000) Integrin signaling's potential for mediating gene expression in hypertrophying skeletal muscle. J Appl Physiol 88: 337-343. doi: 10.1152/jappl.2000.88.1.337
![]() |
[28] |
Blau HM, Pavlath GK, Hardeman EC, et al. (1985) Plasticity of the differentiated state. Science 230: 758-766. doi: 10.1126/science.2414846
![]() |
[29] |
Livak KJ, Schmittgen TD (2001) Analysis of relative gene expression data using real-time quantitative PCR and the 2−ΔΔCT method. Methods 25: 402-408. doi: 10.1006/meth.2001.1262
![]() |
[30] | Zar JH (1999) Biostatistical Analysis. Upper Saddle River NJ: Prentice Hall. |
[31] |
Feng Y, Cao JH, Li XY, et al. (2011) Inhibition of miR-214 expression represses proliferation and differentiation of C2C12 myoblasts. Cell Biochem Funct 29: 378-383. doi: 10.1002/cbf.1760
![]() |
[32] |
Wei X, Li H, Zhang B, et al. (2016) miR-378a-3p promotes differentiation and inhibits proliferation of myoblasts by targeting HDAC4 in skeletal muscle development. RNA Biol 13: 1300-1309. doi: 10.1080/15476286.2016.1239008
![]() |
[33] |
Liu L, Li TM, Liu XR, et al. (2019) MicroRNA-140 inhibits skeletal muscle glycolysis and atrophy in endotoxin-induced sepsis in mice via the WNT signaling pathway. Am J Physiol Cell Physiol 317: C189-C199. doi: 10.1152/ajpcell.00419.2018
![]() |
[34] |
Su Y, Yu Y, Liu C, et al. (2020) Fate decision of satellite cell differentiation and self-renewal by miR-31-IL34 axis. Cell Death Differ 27: 949-965. doi: 10.1038/s41418-019-0390-x
![]() |
[35] |
Ge Y, Sun Y, Chen J (2011) IGF-II is regulated by microRNA-125b in skeletal myogenesis. J Cell Biol 192: 69-81. doi: 10.1083/jcb.201007165
![]() |
[36] |
McCarthy JJ, Esser KA, Andrade FH (2007) MicroRNA-206 is overexpressed in the diaphragm but not the hindlimb muscle of mdx mouse. Am J Physiol Cell Physiol 293: C451-C457. doi: 10.1152/ajpcell.00077.2007
![]() |
[37] |
Farh KKH, Grimson A, Jan C, et al. (2005) The widespread impact of mammalian MicroRNAs on mRNA repression and evolution. Science 310: 1817-1821. doi: 10.1126/science.1121158
![]() |
[38] |
Sayed D, Hong C, Chen IY, et al. (2007) MicroRNAs play an essential role in the development of cardiac hypertrophy. Circ Res 100: 416-424. doi: 10.1161/01.RES.0000257913.42552.23
![]() |
[39] |
Van Rooij E, Olson EN (2007) MicroRNAs: powerful new regualtors of heart disease and provocative therapeutic targets. J Clin Invest 117: 2369-2376. doi: 10.1172/JCI33099
![]() |
[40] |
Van Rooij E, Sutherland LB, Liu N, et al. (2006) A signature pattern of stress-responsive microRNAs that can evoke cardiac hypertrophy and heart failure. Proc Natl Acad Sci USA 103: 18255-18260. doi: 10.1073/pnas.0608791103
![]() |
[41] |
Yang B, Lin H, Xiao J, et al. (2007) The muscle-specific microRNA miR-1 regulates cardiac arrhythmogenic potential by targeting GJA1 and KCNJ2. Nat Med 13: 486-491. doi: 10.1038/nm1569
![]() |
[42] |
Potthoff MJ, Olson EN (2007) MEF2: a central regulator of diverse developmental programs. Development 134: 4131-4140. doi: 10.1242/dev.008367
![]() |
[43] |
Chan JKL, Sun L, Yang XJ, et al. (2003) Functional characterization of an amino-terminal region of HDAC4 that possesses MEF2 binding and transcriptional repressive activity. J Biol Chem 278: 23515-234521. doi: 10.1074/jbc.M301922200
![]() |
[44] |
Liu N, Williams AH, Kim Y, et al. (2007) An intragenic MEF2-dependent enhancer directs muscle-specific expression of microRNAs 1 and 133. Proc Natl Acad Sci USA 104: 20844-20849. doi: 10.1073/pnas.0710558105
![]() |
[45] |
Zhao Y, Samal E, Srivastava D (2005) Serum response factor regulates a muscle-specific microRNA that targets Hand2 during cardiogenesis. Nature 436: 214-220. doi: 10.1038/nature03817
![]() |
[46] |
Van Rooij E, Liu N, Olson EN (2008) MicroRNAs flex their muscles. Trends Genet 24: 159-166. doi: 10.1016/j.tig.2008.01.007
![]() |
![]() |
![]() |
1. | Christopher G Vann, Xin Zhang, Alastair Khodabukus, Melissa C. Orenduff, Yu-Hsiu Chen, David L. Corcoran, George A. Truskey, Nenad Bursac, Virginia B. Kraus, Differential microRNA profiles of intramuscular and secreted extracellular vesicles in human tissue-engineered muscle, 2022, 13, 1664-042X, 10.3389/fphys.2022.937899 |
Moderate Stretch Ratio (n = 7) | High Stretch Ratio (n = 5) | |||
Control | Stretch | Control | Stretch | |
Shift Day 2 | 0.5 ± 0.3 | 0.9 ± 0.5 | 0.7 ± 0.2 | 1.3 ± 0.4 |
Shift Day 4 | 30.8 ± 1.6* | 42.7 ± 4.3* | 28.7 ± 4.5 | 31.2 ± 4.6 |
Shift Day 6 | 47.9 ± 3.0 | 52.1 ± 3.8# | 47.5 ± 3.8 | 39.0 ± 5.3# |
*p < 0.05, Control vs. Stretch; #p < 0.05, Moderate Strain vs. High Stretch Ratio
n = number of independent experiments.